JSTE v48n1 - The STEM Initiative: Constraints and Challenges
The STEM Initiative: Constraints and Challenges
Dennis R. Herschbach
University of Maryland
Abstract
There is considerable national interest in STEM initiatives, but yet there is little discussion concerning what STEM means in terms of a curriculum concept to be applied to school programming. This article focuses on STEM as a curriculum concept. First, STEM programming is discussed in terms of separate subjects, correlated and broad fields curriculum models. The issue of subject structure is examined. A distinction also is made between the four STEM subjects in terms of formal and applicative uses of knowledge. Second, some practical programming issues are discussed. These include the almost exclusive focus on science and math to the exclusion of technology and engineering; the challenge of serving multiple student populations; and the issue of what to do with the “T” in STEM. A concluding section suggests ways that the STEM initiative can be conceptualized in order to realize its considerable potential to achieve curriculum reformulation.
Introduction
Interest in STEM (Science, Technology, Engineering and Math) instructional models is literally exploding across the educational landscape. Universities are exploring STEM models as a way to restructure science and engineering instruction; secondary schools are engaged in experimenting with modified curricula; the educational literature is full of references to STEM initiatives; and consultants and entrepreneurs are rushing into the educational market place with assurances that they too can aid in the implementation of effective STEM programming. Largely initiated and funded by the National Science Foundation, STEM initiatives are now supported by other foundations, professional organizations, universities, publishers, schools systems, and producers of educational materials among groups and individuals that see promise or profit in the possibilities of curriculum reorganization through STEM initiatives ( Kuenzi, 2008 ).
Part of the explanation for the national frenzy over STEM programming is money. Grants from the National Science Foundation in addition to other organizations are funding program experimentation. Scores are jumping onto the money cart to get their share. STEM initiatives feed into a national concern over the relative capacity of the U.S. to compete in the international economic arena. On international tests comparing academic performance, American students do not fare very well. Greater national educational attention on science, technology, engineering and math addresses the political contention that schools must shoulder a good part of the blame for the nation's weakening ability to compete internationally ( Kuenzi, 2008 ; National Academies, 2006 ; The New Commission on the Skills of the American Workforce, 2007 ). But also, powerful national organizations, such as the National Academy of Engineering and the National Academy of Science, are supporting STEM initiatives. There is mounting concern over the lack of young Americans preparing for scientific and engineering professions. ( National Academy of Engineering and National Research Council, 2009 ; Pearson & Young, 2002 ).
STEM does not represent a specific curriculum model; rather, there are many ways to formulate STEM programming. In fact, it is hard to discern what exactly is meant by "STEM." Practically any kind of educational intervention that is even remotely associated with science, technology, engineering or math is referred to as a STEM innovation. This lack of a solidifying perception of STEM threatens over the long-term to destroy support for the movement. Failure to deliver results will probably exceed successes.
Above all, STEM represents a way to think about curriculum change. It is a concept of how to restructure what we teach and what students learn. The purpose of this paper is to first briefly unpack what is meant by STEM in terms of a curriculum concept. What STEM represents is discussed in terms of curriculum theory. Second, some issues related to instructional programming will be explored. By framing the discussion in terms of curriculum theory we can more clearly see some to the constraints and challenges faced as STEM initiatives are pursued. Curriculum theory also helps us to formulate a common framework within which to discuss STEM and its application in the school.
Unpacking STEM as a Curriculum Concept
Traditionally, the most common and widespread curriculum pattern is the separate subjects ( McNeil, 1990 ). Each is taught separately with little attention given to the interrelationships between subjects. Secondary level students, for example, are exposed to discrete subjects to study, such as algebra, chemistry or history. An ends-means curriculum organization tends to be used, starting with pre-specified objectives, or standards, and ending with tests to assess attainment of the discrete course elements. The purpose of instruction is to efficiently transmit a predefined body of formal content thought to be essential to students. The degree to which instruction is "successful" is assessed through tests. Instruction is conceived primarily as a process of knowledge transmission.
In contrast, an implied characteristic underlying STEM is what is termed an "integrated curriculum design." This is a marked departure from the way that instruction tends to be organized and delivered in schools. Subjects such as science, technology, engineering and math are integrated in ways that show more clearly the functional relationship between each ( Kuenzi, 2008 ; McNeil, 1990 ). In real-life situations, knowledge tends to be used across fields of study. The integrated curriculum design attempts to capture the interrelationships within and between subjects and thereby ground learning in the actual way that knowledge is used. Not only is leaning thought to be enhanced, but it is considered to be more relevant. The student learns how knowledge is applied ( McNiel,1999 ; Herschbach, 2009 ).
The Correlated Curriculum
STEM implies an integrated curriculum design. There are two basic ways that integrated curricula are organized: correlated or broad fields. The correlated curriculum pattern tends to be the most poplar option because it retains the identity of each subject, and each may be offered as a separate course ( McNeil, 1990 ). Concepts learned in math, for example, may be applied to physics or technology education through coordinated planning, but each subject area retains its separate identity. It is a more comfortable fit with the ongoing school instructional program because very little adaptation is required to what is already an on-going separate subjects orientation. It is a curriculum pattern that is familiar to administrators, teachers and the educational public. What is required, however, is coordination and planning among the different stand-alone subjects.
One challenge that the correlated curriculum pattern presents is, in fact, the high level of on-going coordination that is required. To be most effective, there has to be a clear relationship between what students learn in one subject with what students learn in the other associated subjects. This requires an ongoing, close working relationship on the part of the involved teachers, with regular and continuing planning and coordination. But in addition, the way that subject fields are formally and "conventionally" organized often has to be abandoned or substantially modified in order to adapt to the requirements of coordinating with the other associated subjects ( McNeil, 1990 ). Algebra instruction, for example, may have to be reorganized and sequenced other than the way that it traditionally has been: little integrated understanding may be achieved if a concept in algebra is presented three months after it is needed in physics and is ignored in engineering.
The Broad Fields Curriculum
The broad fields pattern is a second way to integrate instruction. With the broad fields curriculum, a cluster of related but different subjects is organized into a single area of study ( McNeil, 1990 ). Language arts, graphic communications, and general science are examples. The individual subjects lose their own separate identity since the subject matter from the different fields is combined into a new instructional configuration. A general science course, for example, may include units from biology, physics, earth science, and chemistry. Integration can be done with a single course or with a sequence of related courses.
A fundamental challenge associated with the broad fields curriculum design is to formulate an effective organizing framework for instruction. When the subject matter from different fields is integrated into a new course structure, the structure inherent in the different parent fields tends to be lost. This means that a new way has to be found to organize instruction so that some of the identity of the original parent fields is retained while at the same time an integrated program design is achieved that has a clear organizing framework.
The most common way to achieve a coherent organizing framework is through activities (Figure 1). The curricular emphasis shifts from organizing instruction around the formal structure of fields of study to focusing on a sequence of activities that guide students through the integrated use of knowledge ( Herschbach, 2009 ; National Academy of Engineering and National Research Council. 2009 ). A course, for example, may be organized around the construction and testing of a solar-power vehicle. All of the STEM subjects are brought together to focus on the activity, with knowledge selectively used to address the scientific, engineering and fabrication challenges inherent in designing a solar-power vehicle. Selected formal and applicative knowledge is used (Figure 2). Of course, the conditioning learning factor is the demand the activity makes of the full range of potential knowledge. It is the characteristic of the activity that conditions the extent to which knowledge is used from the different related fields of study ( Mitcham & Mackey, 1972 ).
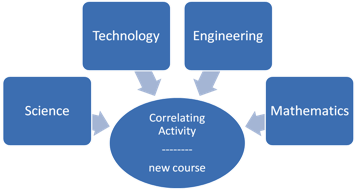
The broad fields curriculum pattern tends to shift instructional focus away from the way that teaching and learning is organized in schools along different discrete subject fields to an activity-based curriculum with less formal identification with traditional fields of study. Formal knowledge is selectively used, but educators are required to think differently about how instruction is organized and taught. The traditional ends-means model of instruction, starting with defined objectives and cumulating in paper and pencil student testing is less appropriate. Progress through content elements tends to be integrative and uneven, not linear, because it is linked with activity. Like the correlated curriculum design, continuing planning and coordination, nevertheless, are required among teachers; but teachers also have to learn to instruct and evaluate students in different ways.
Use of the design process is one of the more common ways that broad fields programming is addressed ( Herschbach, 2009 ; National Academy of Engineering and National Research Council, 2009 ). The design problem functions as a correlating channel for learning, with particular emphasis placed on the integration of science and math with technology and engineering ( Banks, 1994 ; Kolodner, 2002 ; Sanders, 2008 ; Raizen, et al., 1995 ; Wicklein, 2006 ). Students bring what knowledge they know to bear on the design problem, and what they do not know they research. Knowledge is used as a tool to solve problems. At the same time, however, there is room for well-defined, selected stand-alone units of instruction that address the acquisition of formal knowledge.
Subject Structure
As previously suggested, in the case of both the correlated and broad fields patterns, the need to coordinate the sequencing of subjects presents a formidable challenge. It is, ultimately, the formal structure of a given subject that defines its characteristics and sets it off from other subjects. As Bruner (1961) reminds us, helping students to identify and understand the underlying formal "structure" of various fields of study is essential to learning. The focus is on higher-level conceptual learning which gives coherence to what sometimes can be fragmented and loosely organized "bits and pieces" of knowledge. The structure contains crucial concepts that provide order, cohesion and significance to the subject. Bruner (1961) contends “the curriculum of a subject should be determined by the most fundamental understanding that can be achieved of the underlying principles that give structure to that subject” (p. 18).
The formal structure of a field of study can be defined in three ways ( McNeil,1990 ; ">1999 ). One is the organizational structure (Figure 2). This is the way that one subject differs from others and defines the borders and divisions within the subject. The formal structure is what most people are familiar with. At a subtler level are a substantive and a syntactical structure. Substantive structure relates to the kinds of questions framed, the theories applied, and the data used in the course of intellectual inquiry. Syntactical structure relates to the intellectual devices used with subject fields to collect data, test assertions, and generalize findings.
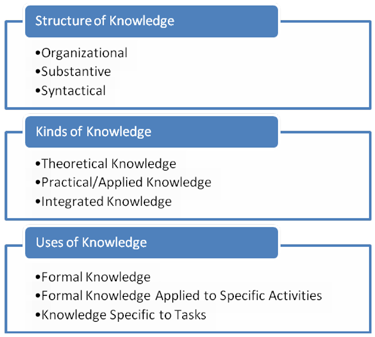
Because structural characteristics are most clearly embedded in specific formal, stand-alone subject areas, instructional stress tends to be placed on a separate subjects organizing pattern in schools ( McNeil, 1990 ; Newman, 1994 ). This is one reason why the separate subject pattern is so widely used for organizing instruction. The formal structure is clear in geometry, physics and chemistry, for example, but considerably less so in technology education, general science or cultural studies. It is more difficult to retain and convey the structural characteristics of a field of study through an integrated curriculum design. Sequencing is a challenge, but also integrated curriculum patterns tend to make selective use of instructional elements within fields of study; instructional identity tends to get lost.
Formal and Applied Knowledge
Another way to think about the formal structure of fields of study is the difference between formal and applied knowledge (Figure 2) that influences how subject matter is selected and sequenced. In fields such as math, physics, and chemistry, as suggested, students tend to engage in learning the formal structure. These are the concepts, laws, theorems and intellectual devices that make up the substantive and syntactical structure of the specific field. They underlie the field and make it distinct. There often is little concern about how formal knowledge is applied, however. In contrast, in fields such as engineering and technology, formal knowledge is used selectively to address specific problems, so only a partial understanding of the formal subject is achieved ( Herschbach, 1996 ). It is applied knowledge, specific and limited knowledge that is needed to only address the current problem at hand. Some concepts in chemistry, for example, simply may not be covered in engineering and math and biology may be overlooked entirely.
Unfortunately, applied knowledge may be considered of lower importance because it relies on only a partial understanding of formal learning. Engaging students in the learning of formal and applied knowledge across four integrated instructional areas, such as in STEM, is a challenge.
Uses of Knowledge
The challenge of addressing the differences between formal and applied knowledge becomes apparent when considering how knowledge is applied to work. The broad fields curriculum pattern is most widely used with technical instruction because it closely mirrors the way that knowledge is selected and applied by practitioners. Engineers, technicians of all sorts, skilled craft workers and a host of other individuals basically use three kinds of knowledge: selected elements of formal knowledge, formal knowledge as it is applied to the specific task, and knowledge specific to the task (Figure 2).
Many work tasks draw from formal knowledge. For example, specific scientific procedures or mathematical concepts may be an integral component of the job task. Selected knowledge basically is applied unaltered in its formal form.
Work tasks also make selective use of formal knowledge applied in conjunction with specific technical knowledge. Knowledge of geometry is needed, for example, to calculate rafter and stud angles on a roof dormer. A combined knowledge of both roof design and geometry is required. The builder needs to learn the selective use of geometry, but does not have to have a complete understanding of the subject field of geometry as it is formally organized.
But there are also some tasks that are purely technical and relate solely to the technical procedure. They are specific to the technical field and do not make use of the formal knowledge of other subjects.
As previously observed, because of the way that the broad fields curriculum pattern selects and makes use of the three forms of knowledge, it is less useful for conveying an understanding of the formal structure of fields such as calculus, physics, chemistry, or biology, among others. On the other hand, the broad fields pattern is a very effective way to organize engineering and technology instruction because they are interdisciplinary and applicative subjects (the T and E in STEM). Instruction tends to be built around the integrated use of knowledge selectively drawn from formal fields. Instruction is organized according how knowledge is used ( McNeil, 1990 ).
But again, this pattern is less useful for the purpose of organizing formal subjects such as science and math because of the difficulty in adequately conveying an understanding of the formal structure of the fields. This disjunction between the two ways that knowledge is organized and used creates complex organizing and programming challenges.
The Character and Validity of Knowledge
As suggested in the above discussion, differences between interdisciplinary, integrative subjects, such as engineering and technology, and formal academic subject fields such as physics and algebra, are a major curriculum stumbling block with STEM initiatives that yet is to be resolved. These issues can be further examined by focusing on fundamental epistemological characteristics that tend to be glossed over, that is, issues relating to the character and validity of knowledge.
"Science" is a broad descriptive term that acquires specificity only when it defines a particular field of study, such as physics, or better still, molecular physics. The function of science is to discover and advance knowledge . To this end, science makes use of the scientific tools of investigation, and relies heavily on mathematics as an analytical tool . Specific fields of study tend to be taught formally as stand-alone subjects. As formal fields of study, science and mathematics have a close symbiotic relationship. Instruction in both fields also tends to convey a broad and deep understanding of the organizational, substantive and syntactical structures of the fields. Indeed, as previously stressed, a structural understanding is essential to learning ( Bruner, 1960 ; Herschbach, 1995 ; McNeil, 1999 ).
The term "technology" is even broader than "science," and refers to just about everything in the designed, man-made world. There is no practical way to convey meaningful technology instruction without tying it to specific activity. Technology is manifested through abstract and concrete artifacts ( Feenberg, 2002 ; Dasgupta, 1996 ; Pacey, 1999 ; Skolimowski, 1966 ). When technology is defined in terms of a specific application, such as micro precision instrumentation, instruction is integrative and interdisciplinary in scope. And it is the bond with application that distinguishes technological knowledge from set bodies of formal knowledge (Figure 2.) Technological applications make use of formal knowledge, but in very specific ways. The inherent interdisciplinary activity makes technology a good candidate for an integrative framework around which STEM subjects can be organized except that only selective use is made of formal knowledge.
"Engineering" differs from the other three subject areas in that it primarily refers to preparation for specific occupations ( Oaks, Leone & Gunn, 2001 ). It is in one sense a vocational subject at the collegiate level. The requirements of the specific occupational field define the instructional content. Engineering, then, like technology, selectively makes use of formal knowledge from science, mathematics and technology . The specific selection and use of knowledge, however, depends on the occupational field of engineering understudy.
Of the four STEM areas, "math" is the most clearly defined as a formal subject. It already has wide recognition in schools, and instruction tends to be organized around students learning its formal organizational, substantive, and syntactical structures. Other STEM subjects tend to supply a supporting role in that they demonstrate how math concepts can be applied with the expectation that better math learning will result. The broad fields curriculum pattern, as previously observed, has limited use since only selected mathematical concepts are applied in a very restricted way to address the particular activities. As suggested, the correlated curriculum design often lacks full integration.
The four STEM fields, in sum, have epistemological characteristics that differ markedly. These characteristics must be fully recognized and accommodated in programming in order to preserve the intellectual integrity of each field. Otherwise a very limited understanding results that undervalues specific intellectual contributions or ignores the collective value of each.
Some Issues Related to Programming
In addition to issues relating to the substance and structure of knowledge STEM as a curriculum concept presents a number of practical programming issues. To be sure, integrated curriculum designs are not new. They emerged during the 1920s as part of the progressive school era (Kilebard, 1987). At the time it was recognized that the intellectual integrity of the various integrated subject fields was in part lost through integration. But educators were primarily concerned with making school instruction more relevant to the life experiences of students. Today, there is an educational environment that is strongly focused on a separate subjects orientation, “academic” achievement, testing, and an emphasis on the “basics.” There is considerably less concern about making instruction more relevant to life. It is difficult so see how integrated STEM programming with such applicative subjects such as technology and engineering fit into current school programming. The tensions between current subject-matter divisions and the integrative programming implied by STEM create a number of programming issues that yet are to be resolved.
The Illusion of STEM Programming
One major issue is the limited perception of what STEM represents. STEM is widely perceived as related mainly to strengthening math and science education ( National Commission on Mathematics and Science, 2000 ). As one recent national report observes, “Despite all of the concerns by policy makers, educators, and people in industry about the quality of U.S. K-12 STEM education, the role of technology education and engineering education have hardly been mentioned. In fact, the STEM acronym has become shorthand for science and mathematics education only, and even these subjects typically are treated as separate entities” ( National Academy of Engineering and National Research Council, 2009 , p. 150). “Technology,” along with applications to engineering is assumed to automatically fall under math and science. Much of the national attention STEM has attained is because of its potential impact on math and science education, with little interest in “retooling” the subject fields in order to share instructional space with technology and engineering ( Kuenzi, 2008 ; Moyer-Packenham, et. al, 2008 ; National Commission on Mathematics and Science Teaching for the 21 st Century (2007).
But even with the focus on math and science, there is little evidence that the programming implications of STEM are realized. One of the most widespread, but highly limited approaches to STEM programming is to retain the traditional subject matter distinctions in school and to imagine that integrated learning is actually happening. When there is an increase in math students, for example, it is assumed that there is an increase in “STEM” students; but yet, it may be hard to find ways that math instruction has been changed. This is largely an exercise in labeling. A benefit may be that greater attention is directed toward math and science, but it is a highly restricted vision of STEM programming.
A great deal of STEM programming in schools today appears to be in the form of units of study interjected into slightly modified, conventional stand-alone courses. Commercial modules and STEM worksheets abound in the market place, yet they often represent little in the form of substantial change. While there are notable exceptions, what is often referred to as STEM courses requires little in the way to creative, integrated programming. STEM implementation tends to be an illusion.
What is the Target Population for STEM Programming?
Connected to a limited perception of what STEM programming implies are issues related to the student populations to be served. Secondary schools tend to program subjects according to potential achievement levels. Students tend to be scheduled based on an assessment of how well they can perform at a given level ( Newman, 1994 ).
In many schools, the STEM initiative tends to be perceived mainly as a way to strengthen stand-alone math and science courses for college-bound students, with less attention give to “lower” programming levels. STEM is viewed as applying primarily to “college caliber” students. It is anticipated that emphasis on STEM (primarily on the S and M) will result in more students enrolling in college preparatory course work at higher performance levels ( National Academies, 2006 ). There appears to considerable less national interest, however, in programming designed to serve the large student population that does not elect to go to a four-year postsecondary institution of any kind ( Cech, 2009 ; Kuenzi, 2008 ).
Approximately 50% of a given student cohort, does not elect to pursue additional education beyond high school, not to count the students who drop out before completion. National discussion concerning the diverse range of student populations that can profit from variations of STEM programming is limited, but yet thinking about STEM has to be broadened to include more than college-bound students if schools are to serve the great number of electricians, warehouse workers, agricultural specialists and craftsmen and technicians of all kinds that also have to be equipped to participate in our scientific and technologically orientated society. There are multiple target populations that can and need to be served ( Cech, 2009 ; The Workforce Alliance, n.d. ).
Even in the case of more college-orientated programming, there is some question about the extent to which integrated STEM courses of any kind eventually will be accepted for college admission purposes. College’s admission officers continue to think in terms of a separate subjects orientation that is emulated by secondary schools in the preparation of students for entrance examinations. Colleges accept credits for APT courses, but have a lesser understanding of and a greater reluctance to give credit to integrated offerings that engage students in the applied uses of science and math. There are APT examinations in physics and algebra, for example, but none for design, technology and engineering classes. Admission officials understand what chemistry is, but they are not sure what technology education means and they are prone not to accept what appear to be “vocational” subjects. It will be difficult to realize the true potential of STEM programming until what constitutes preparation for college entrance is conceived differently.
What to do with the “T” in STEM?
Given the “conventional” way that knowledge continues to be perceived and organized for instruction, one potentially contentious, emerging issue is where will the "T" in STEM be taught. Some science educators think that they teach about technology since much what goes for "science" teaching today is actually applied technology. Practical applications of scientific concepts are used to enhance science learning.
A case also can be made for technology to be taught through engineering ( National Academy of Engineering and National Research Council, 2009 ; Sanders, 2008 ). Much of engineering consists of science and math applied in the service of technological improvement and advancement. Engineering is largely an applied field with its practitioners seeking solutions to "real" technological problems. But if engineering is to be used as an integrative, correlating center of instruction, which particular field of engineering will be used and why? Civil engineering, mechanical, industrial, sanitation, hog production, aeronautics, among a host of others? There is no easy way to make this decision in school programs serving general instructional purposes for students not yet ready to make a specific, perhaps narrow career choice. At the same time, claims that "general engineering processes are taught" are difficult to sustain unless programming is designed to achieve such an objective. With few exceptions, this objective has not been met ( Kelley, Brenner & Piper, 2010 ).
On the other hand, engineering is an ideal place to demonstrate the interdependent relationship between science, technology, and math. Engineering uses science, technology and math to make things. However, public schools tend not to offer engineering as a subject. The occupational field is relatively small, particularly when broken down into specialties. When STEM is too closely defined as pre-engineering education, it faces the possibility of unduly limiting the number of students that are attracted to the subject. Its appeal may be to a relatively small, select group of students. Roughly, only 5 to 6% of high school graduates enroll nationally as college engineering majors ( Deloatch, 2010 ).
The vocational, technical and technology fields of study also make claim to the "T" in STEM. They have traditionally been applicative subjects deeply immersed in uses of technology. The distinction with engineering applications of technology is primarily one of level and objectives of instruction. Engineering tends to incorporate greater use of science and mathematics at a theoretical level, and the field tends to be more focused on the design rather than on the construction and use of artifacts ( Hill, 2004 ; McAlister, 2004 ). In fields such as vocational and technical education, nevertheless, heavy use is made of technology, and considerable integrative instruction is used because technology itself is integrative.
Technology teacher educators in particular see STEM as a means of achieving greater instructional focus in schools. McAlister (2004) , however, in a study of 44 teacher education programs across the country found that few aspiring teachers had the skills needed to effectively address the science, math and engineering elements of STEM. Without a substantial refocusing of technology teacher preparation programs, it is difficult to see how technology education can effectively interface at the school level with engineering content and with science and math.
Finally, as previously suggested, STEM is widely perceived as related mainly to strengthening math and science education. However, this limits its promise as a reforming concept. "Technology" is assumed to automatically fall under math and science along with applications to engineering. This reduces the potential impact of STEM. The value of using science and math to address “real world problems” is lost in the context in which knowledge is used. It is difficult to adequately address the theoretical, practical (applicative) and integrated uses of knowledge in stand-alone courses organized around the formal structure of math and science courses.
Looking Ahead
A yet unfulfilled promise of STEM is to reconceptualize how knowledge is conceived, organized and taught in schools. Within the scientific and engineering communities today there appears to be a rethinking of how knowledge is generated and used. Some of the most striking advancements are made through the combined use of knowledge spanning across traditionally different intellectual fields. More traditional subject fields are being enriched and expanded through the integration of knowledge from other formerly stand-alone subjects to form new combinations of intellectually integrated knowledge that feeds investigation, discovery and understanding. Biology, for example is crossed with physics and engineering; solar heating research is melded with building material research and new construction technology.
More so than in the 1920s, there is a greater understanding today that new forms of abstract and applied knowledge are highly productive and, perhaps, the key to addressing what are some of the most crucial problems facing humankind. There is a considerable rethinking of the way that abstract knowledge is combined, learned and used. The opportunity, however, is not fully recognized to integrate programming through STEM and to tap into the potential to organize, learn and use knowledge in highly productive ways that were formally limited by encasing teaching and learning in “traditional” stand-alone, clearly defined subjects. To more fully realize the promise of STEM programming means to move away from the conventional separate subjects curriculum design pattern. This requires substantial curriculum reformulation.
How can a STEM initiative that is representative of the integrated curriculum design pattern be functionally integrated? At least three conditions must be addressed: a) an integrated curriculum design brings together the subject matter from different fields of study in order to make clear the underlying interrelationships; b) students are exposed to the formal structure of the fields of study through learning experiences that incorporate the organizational, substantive and syntactical structures underlying the use of knowledge; and c) students engage with learning experiences that use formal, specialized and applicative knowledge.
Today, part of the interest in STEM initiatives is the perception that instruction will become more relevant to students. It is alleged that there is a crisis in education because U.S. students lag far behind in international measures of educational progress. STEM initiatives allegedly will help markedly improve student achievement, particularly in math and science. An additional hoped for outcome is that greater student interest in math, science and engineering will result from grounding instruction in ways that use knowledge. Students more readily see in their studies the practical application of knowledge ( Kuenzi, 2008 ).
Expectations for meaningful curriculum reform, however, likely will be largely unrealized unless STEM initiatives are accompanied by significantly different ways to organize and deliver instruction. We are trying to fit STEM into what is basically still a separate subjects orientation to the organization of formal schooling. As we have briefly discussed, neither the coordinated nor the broad fields curriculum patterns are an easy fit with the existing separate subjects orientation and its link with the testing movement. Integrated learning itself implies a selective, irregular and iterative use of knowledge in contrast to the primarily linear, lock step, ends-means, separate-subjects instructional model that cumulates in testing. While the separate-subject curriculum model falls significantly short of tapping the full potential of STEM, we nevertheless have to find better ways to fit STEM into integrated programming.
How do technical orientated subjects in particular, such as vocational offerings in the high school or technology education courses in the middle school fit best within the STEM scheme of instruction? One way is to conceive of the purpose of instruction less as exposure to separate fields of content to be mastered and more as a correlating center of student experiences with the meaningful application of knowledge to activity. The instructional emphasis is on the academic integration of formal knowledge with technical content. Technical activity is used as a way to expose students to the thought processes involved in technical work, to correlate the teaching of other subject matter, and to enlighten students about how knowledge is generated and used. Students are fully exposed to the organizational as well as the substantive and syntactical structure underling knowledge and its use. The intellectual content embedded in activity is considered more important than potential skill-training use, although skill training continues to be a viable objective. An over-riding purpose of instruction is to provide experiences through which students come to terms with how knowledge is formulated and used to address technical applications.
To make the shift from a separate subjects emphasis, however, is a daunting challenge. It will demand new ways to think about schooling, its purpose, and the organization and presentation of instruction. The yet unrealized potential of the STEM initiative is that a new curricular reformulation will emerge that will more effectively expose students not only to the way that formal knowledge is learned but also in ways that it is applied.
Dennis R. Herschbach is an Associate Professor in the Department of Education Policy Studies in the College of Education, University of Maryland, College Park. He can be reached at drhersch@umd.edu .
References
Banks, F. (1994). Teaching technology . London: Routledge.
Bruner, J.S. (1961). The process of education. Cambridge, MA: Harvard University Press.
Cech, S.J. (2009). Career skills said to get short shrift: U.S. seen lagging in melding preparation for college, work. Education Week , 28 (21), 1, 12-13.
Dasgupta, S. (1996). Technology and creativity . New York: Oxford University Press.
DeLoatch, E. (2010). Personal communication, July 2010. (Dean of Engineering, Morgan State University).
Feenberg, A. (2002). Transforming technology: A critical theory revisited . New York: Oxford University Press.
Herschbach, D.R. (2009). Technology education foundations and perspectives . Homewood, IL: American Technical Publishers.
Herschbach, D.R. (1995). Technology as knowledge: Implications for instruction. Journal of Technology Education , 7(1) , 31-42.
Herschbach, D.R. The content of instruction (1996). In C.P. Campbell (ed.), Education and training for work , Volume 1- Planning Programs. Lancaster, PA: Technomic Publishing, 109-131.
Kelley, T.R., Brenner, D. & Pipoer, J (2010). Two approaches to engineering design: Observations in sTEm education. Journal of sTEm Teacher Education , 47(2) , 5-40.
Hill, R.B. (2004). Technology teacher education and engineering design. 91 st Mississippi Valley Technology Teacher Education Conference, Rosemont, IL.
Kolodner, J.L. (2002). Facilitating the learning of design practices: Lessons learned from an inquiry into science education. Journal of Industrial Teacher Education , 39(3) , 9-40.
Kuenzi, J.J. (2008). Science, technology, engineering and mathematics (STEM) education: Background, federal policy, and legislative action. Washington, DC: Congressional Research Service
Kliebard, H.M. (1986). The struggle for the American curriculum 1893-1958 . New York: Routledge.
McAlister, B. (2004). Are technology education teachers prepared to teach engineering design and analytical methods? 91 st Mississippi Valley Technology Teacher Education Conference, Rosemont, IL.
McNeil, J.D. (1990). Curriculum: A comprehensive introduction . Boston: Little, Brown and Co.
McNeil, J.D. (1999). Curriculum: The teacher's initiative . Columbus, OH: Merrill.
Mitcham, C., & Mackey, R. (1972) Introduction: Technology as a philosophical problem. In C. Mitcham and R. Mackaey (Eds.), Philosophy and technology (pp. 1-30). New York: The Free Press.
Moyer-Packenham, P.Ss., Anastasis, K., Johanna, J.B., Faye, H. and Irby, N. (2008). Participation by STEM faculty in mathematics and science partnership activities for teachers, Journal of STEM Education: Innovations and Research , 10(2).
National Academy of Engineering and National Research Council (2009). Engineering in K-12 Education Understanding the Status and Improving the Prospects. Washington, D.D.: The National Academies Press.
National Academies (2006). Rising above the gathering storm: Energizing and employing America for a brighter economic future . Washington, D.C.: Washington, D.C.: National Academic press.
National Commission on Mathematics and Science Teaching for the 21 st Century (2000). Before it is too late . Washington, D.C.: Author.
Newman, J.W. (1994). American teachers . New York: Longman.
Oaks, W.C, Leone, L.L. & Gumm. C.J. (2001). Engineering your future . St. Louis, MO: Great Lakes Press.
Pacey, A.(1999). Meaning in technology . Cambridge, MA: MIT Press.
Pearson, G. & Young, T. (2002). Technically speaking . Washington, DC: National Academy Press.
Raizen, S.A., Sellwood, P., Todd, R.D., and Vickers, M. (1995). Technology education in the classroom: Understanding the designed world : San Francisco: Jossey-Bass.
Sanders, M. (2008). Integrative STEM education: Primer, The Technology Teacher , 68(4), 20-26.
Skolimowski, H. (1966). The structure of thinking in technology. Technology and Culture , 7(3), 371-383.
The New Commission on the Skills of the American Workforce. (2007). Tough choices or tough times . Washington, D.C.: National Center on Education and the Economy.
The Workforce Alliance (n.d.).
Finding the forgotten middle: Middle-skill jobs in America today and tomorrow
. Washington, D.C.: Skills 2 Compete: Author.
www.skills2compete.org
.
Wicklein, R.C. (2006). Five good reasons for engineering design as the focus for technology education. The Technology Teacher , 65(7), 25-29.