JOTS v32n2 - Technological Systems and Momentum Change: American Electric Utilities, Restructuring, and Distributed Generation Technologies
Technological Systems and Momentum Change: American Electric Utilities, Restructuring, and Distributed Generation Technologies
Richard F. Hirsh and Benjamin K. Sovacool
"To draw attention today to technological affairs is to focus on a concern that is as central now as nation building and constitution making were a century ago. Technological affairs contain a rich texture of technical matters, scientific laws, economic principles, political forces, and social concerns."
—Thomas P. Hughes, 1983The American electric utility system has been massively transformed during the last three decades. Viewed previously as a staid, secure, and heavily regulated natural monopoly, the system has shed elements of government oversight and now appears to be increasingly susceptible to terrorist attacks and other disruptions. Overturning the conventional wisdom of the 1960s and 1970s, dependence on large-scale generation plants and high-voltage transmission networks now seems to decrease reliability of the system. The same hardware also subjects utility companies to pollution concerns at a time when environmental sustainability has become a serious political and economic issue worldwide. Clearly, much of the formerly sound thinking about the utility system has been overturned. But new thinking has evolved as well. For example, many people have begun advocating the use of small-scale, decentralized technologies known collectively as distributed generation (DG), which offers hope that the electric utility system may become more resilient and environmentally friendly in the near future.
This article examines the reversal of almost a century of momentum in the electric utility system. To do so, it employs the nonengineering version of the systems approach, which has proven useful for analyzing sociotechnical enterprises. As a subtheme, the article explores longterm and cross-industry trends in the use of technologies; in particular, it notes that industrial use of large-scale technologies, which provide great economies of scale, may have reached limits in certain fields, such that small-scale technologies may have become better suited for use. As another subtheme, the article underscores the importance of nontechnical (i.e., social) action in the development of technologies. In the American utility system, the unintended consequences of an obscure law passed in 1978 spurred deregulatory efforts in the 1990s and the development of commercially viable renewable energy and distributed generation technologies. The encouragement of these environmentally preferable and DG facilities continued during the political chaos that emerged early in the twenty-first century, when utility restructuring became questioned in many states.
Using the Systems Approach to Understand Technological Change
To help understand the historical development of electric utilities, one can fruitfully use the "systems approach" that Thomas Hughes originally developed for the social sciences. In his seminal Networks of Power: Electrification in Western Society ( Hughes 1983 ) and other works, Hughes argues that the generation, transmission and distribution of electricity occurs within a technological system. That system extends beyond the engineering realm, and it includes a "seamless web" of considerations that can be categorized as economic, educational, legal, administrative, and technical. Large modern systems integrate these elements into one piece, with system-builders striving to "construct or ... force unity from diversity, centralization in the face of pluralism, and coherence from chaos" ( Hughes 1987 , 52). If the managers succeed, the system expands and thrives while, simultaneously, closing itself. In other words, the influence on it of the outside environment may gradually recede because the system has expanded its reach to encompass factors that might otherwise alter it ( Hughes 1987 , 52).
Hughes's systems approach also employs the notion of momentum, which Networks of Power describes as a mass of "machines, devices, structures" and "business concerns, government agencies, professional societies, educational institutions and other organizations" that "have a perceptible rate of growth or velocity" ( Hughes 1983 , 15). In fewer words, Hughes defines momentum elsewhere as a "mass of technological, organizational and attitudinal components [that tend] to maintain their steady growth and direction" ( Hughes 1989 , 460). The system's tendency to continue along a given path results from the actions of numerous stakeholders, such as educational and regulatory institutions, the investment of billions of dollars in equipment, and the work and culture of people working within an industry. In concert, these elements promote business as usual and the outward show of a large degree of momentum. Furthermore, Hughes explains that system momentum can be aided through the use of "conservative" inventions, i.e., new technologies that preserve the existing system. Managers of the system obviously prefer to maintain their control of affairs and, while they may seek increased efficiencies and profits, they do not want to see introduction of new and disruptive "radical" technologies. As an example, Internet telephony and cell phones may be viewed today as radical inventions by managers of the traditional wired communications network.
To summarize, Hughes's approach suggests that systems develop through human managers' control of elements to exploit the existing social environment. Systems acquire momentum, and they resist alteration as they mature. Momentum, however, is not the equivalent of determinism (which holds that either social or technical factors are exclusively responsible for technological development) or autonomy (which suggests that technologies achieve a level of action independent of human contexts). Rather, momentum in systems—even those having high momentum—can sometimes be altered through "a confluence of contingency, catastrophe and conversion," as Hughes puts it ( Hughes 1989 , 470-471). Through their institutions and actions, humans may play a role in changing momentum, difficult as that may appear in many situations. But such was the case in the American electric utility system.
Origins and Growth of Momentum in the Electric Utility System
The electric utility system began to gain momentum early in the twentieth century. It did so largely as power company managers took advantage of incrementally improving technology. Adopting alternating-current (AC) transmission and steam-turbine technologies, early utility entrepreneurs quickly found they could emulate trends in other industries, most notably by increasing the scale of their business. Economist Alfred Chandler (1977) has shown how the railroad industry served as the epitome of modern enterprises that exploited new technologies to expand their scale and scope. In the process, railroad companies eliminated regional competition and gained panoptic political and economic power, while also earning the contempt of many of their customers. Similarly, through the use of transformers made available in the late 1880s, AC transmission allowed companies to distribute high-voltage power over long distances. And in the first decade of the twentieth century, utility managers began employing compact steam turbines (connected to generators) as prime movers that further encouraged centralization, because the new machines offered tremendous economies of scale. Put simply, as the turbines produced larger capacities of power, the unit cost of electricity declined over a wide range of output. Taking actions that would be imitated by other electrical entrepreneurs, Samuel Insull embraced alternating current and steam turbines for his small Chicago Edison Company. When he took over the firm in 1892, Chicago claimed 20 competitive electric supply firms. By 1907, Insull employed the new technology and consolidated them into the renamed "Commonwealth Edison Company," a virtual monopoly in the city and its environs ( Hughes 1983 , 208).
Steam turbine-generators and alternatingcurrent technologies became the core conservative technologies of the utility system, allowing it to produce increasing amounts of power at lower unit cost. Developed for utility use by companies such as Westinghouse and General Electric, the technology showed improvements mainly in two criteria. First, manufacturers designed steam turbines using new metal alloys that could endure higher temperatures and pressures, thus yielding better thermal efficiencies. While Thomas Edison's pioneering 1882 power station (which used reciprocating steam engines) converted just 2.5 percent of a raw fuel's energy into electricity, steam turbines improved over the years to achieve a conversion efficiency of about 40 percent in the 1960s, though the average plant demonstrated an efficiency of about 33 percent (Figure 1). At the same time, manufacturers exploited new knowledge about metallurgy to produce turbines and generators that became ever-larger and took advantage of economies of scale. Following installation of a 5-megawatt (MW) steam turbine in 1905, for example, Samuel Insull's firm procured 12-MW machines in 1911 ( Insull 1915 , 430). More powerful equipment followed: Insull's company employed a 208-MW unit in 1929. After the Great Depression, other American utilities bought units that reached 1,000 MW in 1965 and 1,300 MW in 1972.
![]()
The growth in scale of electric utility technology parallels trends in other industries. As in the manufacturing of automobiles and consumer durables and in service industries and sanitation, managers employed technologies that reduced unit costs, increased efficiency, and added to profitability as the scale of operations increased ( Melosi 2000 ; Noble 1999 ). Some of the scale efficiency occurred for "simple" laws of mathematics and engineering. For example, as the circumference of a pipe that feeds a steam turbine increases by a factor of three, the volume of steam passing through it increases by a factor of nine. In other words, for a modest increase in material inputs (and costs), the industrialist obtains disproportionately large outputs ( Hirsh 1989 , 41). More gains emerge when realizing that many other costs decline as scale increases. Instead of employing two small steam turbines, along with the associated equipment and personnel needed to operate them, a utility could build one large turbine. The power output is equivalent, but at lower unit cost.
Because of associated improvements in transmission, distribution, and control equipment, the use of more fuel-efficient and larger turbine-generators boosted the industry's total factor productivity—a measure of overall operating efficiency ( Kendrick 1961 ; Kendrick 1973 ). In everyday terms, higher productivity meant declining costs and prices. While residential customers in 1892 paid about 544 cents per kilowatt-hour (kWh) (in adjusted 2004 terms), they only paid 10 cents for the equivalent amount of electricity in 1970 ( Edison Electric Institute 1988 ; Edison Electric Institute 1997 ; U.S. Department of Energy 2005a ). Since lower prices and the availability of attractive electrical appliances stimulated demand, consumption jumped at a 12 percent annual growth rate from 1900 to 1920 and at a 7 percent average annual rate from 1920 to 1973 ( Landsberg and Schurr 1968 ).
While the use of conservative, incrementally improving technologies contributed to the utility system's growing momentum, so did nontechnical elements. By focusing attention on such elements, Hughes's system approach helps us understand that technology evolves in a social milieu. Perhaps most surprisingly, the creation of regulatory oversight of the utility industry contributed significantly to momentum. As academics and politicians began to realize during the Progressive era in American politics, which lasted from about 1896 to the beginning of World War I, certain types of businesses appeared unable to operate within the traditional competitive market environment: they required construction of capital-intensive facilities that limited the number of rivals to those few that could secure financing. Meanwhile, competition among firms had already led to political corruption in attempts to win franchise rights from city leaders. These businesses, such as railroads and the increasingly large electric power companies, constituted "natural monopolies"—businesses that offered services most efficiently and cheaply only if they remained free from competition ( Hirsh 1999 , 17-18). Regulation became viewed as a popular approach for gaining the benefits of large-scale companies while protecting the public from potential abuses. In other words, the existence of natural monopolies seemed to call for the regulatory commission as a new, socially valuable institution.
While casual observers might assume that industrial tycoons would resist government regulation, Samuel Insull and other prescient managers realized that regulation actually could help them to consolidate a fledgling industry. As early as 1898, Insull argued that government oversight would confer legitimacy to power companies as natural monopolies and, as important, it would enable utilities to reduce their cost of financing by lessening the risk incurred by investors. Regulation would also protect utilities from attempts at municipal takeovers ( Insull 1915 , 34).
Government oversight of utilities added to system momentum, ironically by giving utility managers a large measure of control. Because regulators guaranteed an almost certain income stream and financial viability, utilities benefited. Moreover, regulation offered the appearance of watchful supervision without really providing much, thereby providing a large degree of clout to utility managers. With the end of the Progressive era, state regulators received little attention from the news media or state legislators, which provided few resources to combat the utilities' well-paid lawyers and consultants. Meanwhile, utility managers courted regulators, and they publicly exaggerated the positive role that regulators played in maintaining a successful large-scale enterprise. Regulators did little to disabuse the public of this notion. They happily went along with the charade because they enjoyed the little prestige and power they acquired by being associated with an industry that provided declining prices for an increasingly necessary commodity ( Hirsh 1999 , 41-46). In short, utility managers had "captured" the regulatory apparatus, which contributed to their control of a system that had developed growing momentum ( Stigler 1971 ; McCraw 1984 ).
Beyond regulators, utility managers won the support of other stakeholders who sought to keep the system moving in the same direction and with continuously increasing mass and velocity. Investment bankers constituted one supportive party as they profited from supplying money for the capital-intensive industry. They also helped create holding companies, which offered power company managers access to financial resources and professional management expertise. Manufacturers of electrical equipment further contributed to momentum because they flourished monetarily when utility companies expanded and required more advanced technologies. To train utility executives and middle-managers, educational institutions (starting with MIT and Cornell University) fashioned degree programs for electrical engineers and also became tacit contributors of momentum. Utility customers, meanwhile, appeared happy, as electrification benefited people with higher material standards of living and a sense of social progress ( Hirsh 1989 , 26-35). They did little to impede the growing momentum and, through their increasing purchases of electricity, added to it.
By the 1920s (and continuing into the 1960s), the utility system had gained a huge amount of momentum. Serving as an integral part of the infrastructure that enhanced industrial productivity and made the "good life" possible, the system employed incrementally improving and conservative technologies. Moreover, utility managers gained control of the system by capturing the regulatory framework and by winning support from financial, industrial, educational, and consumer stakeholders—all of whom seemed to reap tangible benefits ( Hirsh and Serchuk, 1996 ). On the surface, it appeared that little could alter the system's trajectory.
Momentum Change Begins
The first challenge to continuously building momentum in the electric utility system arose in the 1960s and 1970s. In a process that has been called "technological stasis," the industry witnessed the apparent end of productivity-enhancing technological improvements. For a host of managerial and technical reasons, the thermal efficiency of steam turbine-generators stopped improving. Though some plants reached efficiencies as high at 40 percent, they also proved to be highly unreliable, which made them unattractive to managers who sought to keep plants online as long as possible. As bad experience grew with these highly efficient plants, managers stopped ordering them, remaining satisfied with the less-efficient, but more trustworthy units ( Hirsh 1989 , 89).
![]()
Soon thereafter, technological stasis occurred again, this time as utilities sought to build ever-larger, higher-capacity power plants. As units grew to exceed 1,000 MW in the 1960s and 1970s, utilities also discovered operational problems as turbine blades suffered distortions and as furnace problems and other defects hampered the units' performance (Figure 2). Despite the desire to attain increased economies of scale, especially in light of the industry's inability to reach higher thermal efficiencies, managers stepped back from the largest units and settled for smaller, proven units. The problem became increasingly serious in light of the 1973 "energy crisis," which caused fuel to become more expensive. In previous decades, the improvement of power-producing technologies had mitigated increased costs in building materials and labor, allowing the industry to reduce the price of its product. But because of technological stasis— along with greatly increased borrowing rates for an industry that consumed vast amounts of capital—power companies soon found themselves uncharacteristically requesting rate hikes from utility commissions ( Hirsh 1989 , 111). Additionally, the higher prices created antipathy among customers who previously supported the structure of the utility system. More tangibly, higher prices motivated customers to purchase less power: for a few years after the onset of the crisis, electricity consumption actually grew at negative rates, while the longer term annual growth rate (from 1973 to 2003) dropped to about 2.4 percent (Figure 3).
Politics and System Momentum
The havoc on the American economy wreaked by the energy crisis spurred policy makers to take action. On the federal level, President Carter made energy policy his first major initiative. Among a set of five laws proposed by Carter and passed by Congress (albeit in greatly diluted form), the Public Utility Regulatory Policies Act (PURPA) of 1978 had the most far—reaching—and least intended—consequences for power companies. It spurred creation of radical technologies; it began the process of deregulation; and it challenged the control held by power company managers. In the process, the law helped change the momentum of the utility system.
Superficially, PURPA appeared to pose little threat to utility companies. But one obscure portion of it offered incentives for the use of efficient cogeneration power plants. These small units often produced less than 100 MW of power—about 10 percent of what utility-owned nuclear and fossil units churned out—and they burned coal, natural gas, garbage, or biomass. The resulting heat first produced electricity using a traditional steam turbine. But instead of dumping the low-pressure steam into the environment, as was common practice for utility power plants, cogenerators employed it for industrial processes. In other words, small cogeneration plants obtained double duty from raw fuel in the form of two valuable products (electricity and process steam), yielding an overall thermal efficiency rate of 50 percent or higher. (Compare this figure to the 40 percent optimum achieved by utility power plants.) Because of this feat, cogeneration plants frequently matched the economic viability of utility units and became increasingly popular, even at their relatively puny scale. Growing from a small percentage of American electric capacity in 1980, cogeneration facilities accounted for 39 percent of capacity in 2002 ( Electric Power Supply Association, 2005 ).
![]()
A related provision of PURPA also spurred research on environmentally preferable technologies that used water, wind, or solar power to produce electricity. More successful than anyone originally anticipated, PURPA prompted work that cut the cost of power produced by solar photovoltaic panels by about 70 percent between 1980 and 1995 ( Sheer 2001 ; Clayton 2004 ). More significantly, it contributed to work that lowered the cost of power produced by wind turbines; by 2002, the average cost of wind-produced electricity dropped to under 5 cents per kWh, a cost that compared favorably with electricity produced by conventional utility plants burning natural gas or coal ( Smith 2004 ; American Wind Energy Association 2005 ).
These smaller-scale generation technologies challenged the established paradigm of the utility industry (and many other industries) that previously relied on large-scale equipment to produce economies of scale. Now, it appeared, power plants producing modest amounts of electricity proved economically viable. Moreover, since they were smaller, they required less time to build, and they put less capital at risk during a period of rapid price inflation. Finally, they matched the slower growth rate of consumption more appropriately: with growth rates remaining under 3 percent per year, consumers needed smaller increments of power to match their demand. Had utilities continued to build their traditional behemoths, huge chunks of power would remain unused when the plants were completed. Small scale, indeed, looked beautiful.
In the parlance of Hughes's approach, these small-scale technologies constituted radical technologies that helped alter the utility system's momentum. They did so by enabling competition for the traditional power companies—at least in the generation sector—and by eroding the control held by utility managers. No longer the sole producers of electricity, power company executives watched as industrial firms (employing cogeneration facilities) and renewable energy entrepreneurs sold competitively priced electricity.
This unintended experiment with competition suggested to influential regulators and legislators in the 1980s that more competition would benefit stakeholders in the electric utility industry. (Not coincidentally, competition had already begun in the airline, telecommunications, and natural gas industries.) Some academics and politicians wondered if utility regulation still had merit, seeing that a traditional justification of government oversight—the fact that power companies constituted natural monopolies—no longer appeared valid. After all, if nonutilities could produce power as cheaply as could utilities, then the big power companies no longer deemed recognition as natural monopolies. And if they were not natural monopolies, they no longer deserved special status as noncompetitive entities that required regulation ( Hirsh 1999 , 119-142). Why not permit increased competition to thrive outside the realm of the PURPAinspired generation companies?
Already feeling their control of the system threatened by pressures resulting from implementation of PURPA, utility managers had more to fear. After the Gulf War focused attention again on the cost and security of energy supplies, Congress passed the Energy Policy Act of 1992. The legislation sought to employ competitive forces to increase domestic fuel production and to improve the efficiency of energy use. One provision gave states the option of opening up their transmission network to use by competitors. The network would serve as a common carrier so any electricity producer could sell power to any customer. Essentially, the law gave states the right to begin competition on the retail level. During the late 1990s, several states (with California being among the first) passed legislation that established competitive retail frameworks for power. By September 2001, 23 states (and the District of Columbia) had passed similar legislation, while regulatory bodies in several other states had reduced their oversight and had introduced market forces into the system.
The restructuring process, stimulated by PURPA and pursued by advocates of market forces, meant that momentum in the utility system had been altered, largely because utility managers lost control of "their" system. For almost a century, managers commanded the huge-scale, incrementally improving conservative technologies that produced and distributed electricity. But in recent decades, they began facing competition from entrepreneurial companies that employed small-scale fossil fuel and renewable energy technologies. In 1992, nonutility companies controlled 1.5 percent of the nation's total power capacity; that number rose to 34.7 percent in 2003 ( U.S. Department of Energy, 2005b ). In addition, power company managers lost the benefit of traditional regulation, which protected companies from competition on the basis of their claim—now challenged— to natural monopoly status. At the same time, managers lost support from those stakeholders who previously buttressed them: financiers, equipment manufacturers, and educational institutions adapted to the changing environment and found new opportunities serving the needs of the growing number of nonutility companies. Employing the terms of the systems approach, then, the sociotechnical elements that had previously contributed to utility system momentum no longer had the same speed nor direction.
Assuming some of the political and economic power that managers once held, other stakeholders began making new waves. Environmental advocates, for example, gained impressive standing in the legislative process that led to creation of restructuring laws. While supporting deregulation in general, they fought for (and in many cases won) provisions in laws that guaranteed funding for renewable energy and energy-efficiency initiatives. In California's version of restructuring, for example, utilities earned the right to receive payment from customers for building what turned out to be expensive power plants during the era of regulation, but which would have little economic value in an era of competition (called "stranded" assets). But environmental advocates won provision for expenditures on energy efficiency work, renewable energy technologies, and for development of research on new technologies that had not yet shown commercial viability ( Hirsh 1999 , 258).
The Promise of Decentralized Electricity Generation
As stakeholders began renegotiating their positions in an altered utility system, the California electricity crisis of 2000 and 2001 created a sense of chaos. Subsequent blackouts in the Midwest during 2002 and in the Northeast and Canada during 2003 contributed further to that disharmony. In California, where one utility declared bankruptcy as a result of the crisis, the state suspended competition altogether and expanded its control of the wholesale market. Such events caused policy makers in other states to reconsider their previous enthusiasm for restructuring and to slow down plans to introduce market forces. At the same time, the Federal Energy Regulatory Commission proposed new initiatives in response to the 2003 blackout that may give it greater control over the increasingly fragile-looking transmission grid. That grid has witnessed serious underinvestment since the 1990s as utility companies and nonutility entrepreneurs remained concerned in an uncertain policy setting about how the grid will be employed and which stakeholders will profit from its use ( Rigby 2003 ).
The unsettled state of affairs in the power system has provided opportunities for advocates of environmentally-friendly and distributed-generation technologies. Taking advantage of the flux within the utility system, especially in states with strong traditions of politically astute environmental advocates, activists won passage of laws for funding of renewable energy and small-scale generation technologies. Customers paid into "public benefit funds" (also known as "system benefit funds") regardless of which company (a traditional utility or nonutility company) provided them with electricity ( Clean Energy States Alliance, 2004 ). And in eighteen states (plus the District of Columbia), advocates convinced legislators to enact laws creating "renewable portfolio standards" that required all competitive power companies to produce (or to purchase) a certain amount of power coming from small-scale, renewable resources. The laws created a market for environmentally preferable technologies and spurred research and development of them so they become more economically viable even without government incentives. In Texas, a law (signed by Governor George W. Bush in 1999) required 2,000 MW of renewable energy to be constructed by 2009 ( Wiser and Langiss, 2001 ). By the end of 2004, producers had already installed 1,293 MW, most of it consisting of competitively priced wind turbines ( Garman 2005 ).
The state-supported move toward smallscale generation technologies reflects the erosion of the conventional paradigm of providing power through large, centralized power generators. Traditionally, policymakers emphasized using the discipline of power engineering to focus on expanding generating capacity to meet aggregated demand at the lowest cost. This approach relied on large plants because utility managers believed that bigger facilities offered lower fixed costs and were better driven by economies of scale ( Capehart, Mehta, and Turner 2003 ; Lovins et al. 2002 ).
By contrast, an emerging paradigm of distributed generation emphasizes decentralized, modular, and on-site production of electricity. Proponents of DG argue that small, local plants (often having capacities of between 5 and 30 MW) are frequently less expensive, more efficient, more reliable, more flexible, and less damaging to the environment than large utilityowned power plants that burn fossil fuels. Since many renewable energy technologies—such as wind turbines and photovoltaic systems—are smaller and decentralized, they serve as examples of DG technologies. Even generation equipment that employs fossil fuels, such as natural gas and coal, can be considered valuable DG resources, especially when they exploit waste heat generated through combustion or fuel conversion. These comprise cogeneration plants (also known as combined heat and power [CHP] facilities) like those encouraged by PURPA. They may also include very small steam turbines known as micro-turbines that produce power in the range of 25 to 500 kW while also recycling waste steam for water or space heating needs ( Capehart 2003 ). Phosphoric acid fuel cells have become popular too. Being pursued by public benefit funding programs in some states, the devices (which are being tested in 200 kW to 11 MW sizes) take in hydrogen-rich fuel and create electricity through a chemical process rather than by combustion, yielding few particulate wastes ( Yacobucci and Cutright, 2004 ). The process also generates heat, which can be used as a profitable by-product. Beyond these DG technologies that offer high efficiencies are modular internal combustion engines that run on diesel fuel or gasoline. In their smallest incarnations (about 1 kW), they provide backup power for homes and recreational vehicles. In larger increments, they supply power for hospitals and commercial venues. Generally speaking, DG technologies can be employed in isolated "islands"—independent of the transmission grid—or attached to it, in which case they serve as small power units that contribute power to it.
Consumer advocates who favor DG point out that distributed resources can improve the efficiency of providing electric power. They often highlight that transmission of electricity from a power plant to a typical user wastes roughly 4.2 to 8.9 percent of the electricity as a consequence of aging transmission equipment, inconsistent enforcement of reliability guidelines, and growing congestion ( Silberglitt, Ettedgui, and Hove 2002 ; Lovins 2002 ). At the same time, customers often suffer from poor power quality—variations in voltage or electrical flow—that results from a variety of factors, including poor switching operations in the network, voltage dips, interruptions, transients, and network disturbances from loads. Overall, DG proponents highlight the inefficiency of the existing large-scale electrical transmission and distribution network. Moreover, because customers' electricity bills include the cost of this vast transmission grid, the use of on-site power equipment can conceivably provide consumers with affordable power at a higher level of quality. In addition, residents and businesses that generate power locally have the potential to sell surplus power to the grid, which can yield significant income during times of peak demand.
Industrial managers and contractors have also begun to emphasize the advantages of generating power on site. Cogeneration technologies permit businesses to reuse thermal energy that would normally be wasted. They have therefore become prized in industries that use large quantities of heat, such as the iron and steel, chemical processing, refining, pulp and paper manufacturing, and food processing industries ( International Energy Association 2002 , 41-49). Similar generation hardware can also deploy recycled heat to provide hot water for use in aquaculture, greenhouse heating, desalination of seawater, increased crop growth and frost protection, and air preheating ( Kleinbach and Hinrichs 2002 , 311).
Beyond efficiency, DG technologies may provide benefits in the form of more reliable power for industries that require uninterrupted service. The Electric Power Research Institute reported that power outages and quality disturbances cost American businesses $119 billion per year ( Hinrichs, Conbere, and Lobash 2002 ). In 2001, the International Energy Agency ( 2002 ) estimated that the average cost of a one-hour power outage was $6,480,000 for brokerage operations and $2,580,000 for credit card operations (44). The figures grow more impressively for the semiconductor industry, where a twohour power outage can cost close to $48,000,000 ( Lin 2004 ). Given these numbers, it remains no mystery why several firms have already installed DG facilities to ensure consistent power supplies.
Perhaps incongruously, DG facilities offer potential advantages for improving the transmission of power. Because they produce power locally for users, they aid the entire grid by reducing demand during peak times and by minimizing congestion of power on the network, one of the causes of the 2003 blackout ( Congressional Budget Office 2003 ). And by building large numbers of localized power generation facilities rather than a few large&mdsah;scale power plants located distantly from load centers, DG can contribute to deferring transmission upgrades and expansions—at a time when investment in such facilities remains constrained ( International Energy Agency 2002 ; Pepermans 2003 ). Perhaps most important in the post- September 11 era, DG technologies may improve the security of the grid. Decentralized power generation helps reduce the terrorist targets that nuclear facilities and natural gas refineries offer, and—in the event of an attack— better insulate the grid from failure if a large power plant goes down ( Friedman and Homer- Dixon 2004 ).
Environmentalists and academics suggest that DG technologies can provide ancillary benefits to society. Large, centralized power plants emit significant amounts of carbon monoxide, sulfur oxides, particulate matter, hydrocarbons, and nitrogen oxides ( Kleinbach and Hinrichs 2002 , 249). The Environmental Protection Agency has long noted the correlation between high levels of sulfur oxide emissions and the creation of acid rain. Because they concentrate the amount of power they produce, large power plants also focus their pollution and waste heat, frequently destroying aquatic habitats and marine biodiversity ( Kleinbach and Hinrichs 2002 , 307-308). On the other hand, recent studies have confirmed that widespread use of DG technologies substantially reduces emissions: A British analysis estimated that domestic combined heat and power technologies reduced carbon dioxide emissions by 41 percent in 1999; a similar report on the Danish power system observed that widespread use of DG technologies have cut emissions by 30 percent from 1998 to 2001 ( International Energy Association 2002 , 92). Moreover, because DG technologies remain independent of the grid, they can provide emergency power for a huge number of public services, such as hospitals, schools, airports, fire and police stations, military bases, prisons, water supply and sewage treatment plants, natural gas transmission and distribution systems, and communications stations ( Liss 1999 ). Finally, DG can help the nation increase its diversity of energy sources. Some of the DG technologies, such as wind turbines, solar photovoltaic panels, and hydroelectric turbines, consume no fossil fuels, while others, such as fuel cells, microturbines, and some internal combustion units burn natural gas, much of which is produced in the United States. The increasing diversity helps insulate the economy from price shocks, interruptions, and fuel shortages ( Herzog, Lipman, and Edwards 2001 ).
Although estimates vary, total-customer owned generation of distributed resources remains comparatively small, although it is expected to grow rapidly. The Energy Information Administration classified only 0.5 percent of electricity generation in 2000 as "nonutility" generation while noting that cogeneration systems in industrial sectors produced 3.6 percent of electricity for their own use ( Congressional Budget Office 2003 ). However, an Electric Utility Consultants (2004) study concluded that distributed generation units—classified as decentralized generators between 1 and 60 MW—provide an aggregate capacity of 234 gigawatts (GW) through approximately 12.3 million units. (The total capacity of American power plants in 2003 was 948 GW). The American Gas Association (2000), meanwhile, predicted that renewable energy technologies will provide over 15 percent of electricity in the United States by 2020, and the U.S. Department of Energy's Energy Information Administration (2000) projected that distributed generation technologies will likely surpass 25 percent of electricity generation by the same year.
Regardless of these projections, proponents of distributed generation caution that DG technologies are not designed or intended to replace the power grid. The United States Combined Heat and Power Association notes that DG facilities could not reliably serve as substitutes for all large, centralized power plants since they cannot meet all customers' needs (especially customers in urban areas with severe land, permitting, and citing constraints) ( Fallek 2004 ). The American Wind Energy Association (2004) adds that wind turbines will primarily augment, rather than replace, the grid.
As compelling as DG appears, the transition to a new paradigm that employs it widely will not necessarily occur smoothly due to a host of social, technical, and political impediments. By far the largest social constraint consists of the belief that renewable energy systems and decentralized units have higher operating costs than large centralized facilities. As a result, many policymakers and consumers believe that renewable technologies have not become cost competitive with traditional fossil fuel sources of electricity ( Pepermans 2003 ; Colorado Renewable Energy Society 2002 ). In addition, the financial payback period for renewable energy systems still appears too long for fiscally conservative customers. Businesses often look for two to three year timeframes for obtaining a positive cash flow after making capital investments. Yet wind turbines require about six to nine years for payback, and their installation sometimes suffers from long project lead times, lack of suitable service infrastructure, and poorly developed sales channels ( Rendon 2003; Liss 1999 ).
The biggest technical impediment consists of connecting DG technologies to the grid. This interconnection challenge really constitutes a set of related smaller problems: voltage control (keeping voltages within a certain range), the balancing of reactive power (using power to regulate the flow of alternating current to maintain proper grid synchronization), and safety (ensuring the adequate protection of people working on the grid) ( International Energy Agency 2002 , 73-75). Currently, those using DG technologies often depend on custom-designed electronics packages to solve these problems. The great expense in developing such packages obviously creates a disincentive for new users ( Ostergaard 2003 ; Pepermans 2003 ).
Perhaps more significant are the challenges relating to regulatory and political inertia within the United States. Lingering monopoly rules and discriminatory rate structures still create political obstacles towards investing in DG technologies. Customers who seek to use DG often face high exit fees imposed by utilities that seek to recover stranded costs. Further giving an unfair advantage to existing producers, utilities and other owners of old coal-fired power plants have won exemption from 1970 Clean Air Act. These "grandfathered" plants can often operate more cheaply than any type of new generation technology. They therefore enjoy an artificial advantage over some DG technologies, which must meet current and future environmental standards ( Casten 1998 , 203; Meyer 2003 ).
Conclusion
The tentative move toward DG represents a somewhat paradoxical return to the electric utility industry's roots. Thomas Edison inaugurated the industry in 1882 when he supplied direct-current (DC) power to a host of nearby businesses in the financial district of New York. Because DC power could not be transmitted over long distances, Edison hoped to sell smallscale generation equipment to commercial customers (such as hotels and industrial firms) that had large demands for electricity. Individual homeowners would be served by small power stations like Edison's original, which would be dotted throughout cities at regular intervals. This model, of course, became displaced by one that employed large centralized power stations to create huge amounts of power sent over alternating- current transmission networks. Overcoming the problem of limited distance distribution posed by Edison's DC arrangement, the AC approach lent itself better to the economies of scale that became apparent in generating equipment over the next eight decades.
But even consideration of a return to the use of distributed generation resources highlights the significant change in momentum that has occurred within the electric power system. Unlike in the period from the early twentieth century to the 1970s, the technological basis for the system remains in flux today: large-scale generation and transmission technologies no longer offer prized economic benefits, and they recently have begun to be seen as security and reliability threats to a society that increasingly depends on electricity. The experience contains parallels with other industries that once valued large scale. In the steel, biotechnology, agriculture, microelectronics, pharmaceutical, and mining industries, companies have recently employed small-scale technologies to gain increased flexibility, security, and lower costs.
The utility system's momentum has been altered by more than the shift away from traditional large-scale technologies. It has also been changed by those who wield economic and political power. As utility managers lost the benefit of state regulation, which previously guaranteed their companies' status as natural monopolies, they found themselves competing with entrepreneurs who employed small-scale technologies. Simultaneously, other supporting stakeholders (i.e., financiers, equipment suppliers, academic institutions, and politicians) abandoned their former allies, realizing that they could benefit from making alliances with new players in the system. As utility managers lost control over the system, new players, such as environmental advocates and policy makers, sought to advance their own (and different) visions of a utility paradigm. During the chaotic first years of the twenty-first century, the old and new stakeholders have not yet reached consensus for a new paradigm for the utility system. In such an untidy environment, advocates of distributed- generation technologies have seen opportunities to redirect the system's momentum.
If nothing else, this study of the American electric utility system demonstrates that systems with momentum do not carry that momentum indefinitely. Evolving systems are not, as Hughes (1983) pointed out, "driverless vehicles carrying society to destinations unknown and perhaps undesired" (462). Rather, human stakeholders play important roles in channeling momentum. Though they may not always realize the consequences of their actions—contributing to forces that alter momentum in unanticipated ways—people remain at the core of technological systems because of their concern for political control, influence, money, and power. This conclusion suggests the value of the systems approach for teaching about technology and society in school at several levels. In particular, the approach reveals the subtle and explicit interdependency of hardware and nontechnical elements in modern society.
Richard F. Hirsh is a professor of history and science & technology studies at Virginia Tech in Blacksburg, Virginia.
Benjamin K. Sovacool is a PhD student in science & technology studies at Virginia Tech, Blacksburg, VA.
Acknowledgment
The authors are grateful to the National Science Foundation for grants DIR-9012087, SBR- 9223727, SES-0112430, and ECS-0323344, which have supported elements of the work reported here. Any opinions, findings, and conclusions or recommendations expressed in this material are those of the authors and do not necessarily reflect the views of the National Science Foundation.
References
American Gas Foundation. (2000). Fueling the Future: Natural Gas and New Technology for a Cleaner 21st Century , at http://www.fuelingthefuture.org/contents/NaturalGasPowersUp.asp .
American Wind Energy Association. (2004). "Distributed Generation: Revolutionizing the Grid," at http://groups.msn.com/AAEA/utilities.msnw .
wind05 American Wind Energy Association. (2005). "Wind Energy Costs," http://www.awea.org/faq/tutorial/wwt_costs.html .
Capehart, Barney L., Paul Mehta, and Wayne Turner. (2003). "Distributed Generation and Your Energy Future." Cogeneration and Distributed Generation Journal 18(4): 17-33.
Capehart, Barney L. (2003). "Micro-turbines." Whole Building Design Guide (August), http://www.wbdg.org/design/microturbines.php .
Casten, Thomas R. (1998). Turning Off the Heat: Why America Must Double Energy Efficiency to Save Money and Reduce Global Warming . New York: Prometheus Books.
Chandler, Alfred D. (1977). The Visible Hand: The Managerial Revolution in American Business . Cambridge, MA: Harvard University Press.
Clayton, Mark. (2004). "Solar Power Hits Suburbia." The Christian Science Monitor (February 12), 14.
Clean Energy States Alliance. (2004). "CESA Year One: A Report on Clean Energy Funds in the U.S., 2003-2004," at http://www.cleanenergystates.org/ .
Colorado Renewable Energy Society. (2002). "Renewable Portfolio Standard Backgrounder." February 4, www.cres-energy.org/documents/RPS%20Backgrounder_MW-2-04-02.pdf .
Congressional Budget Office. (2003). Prospects for Distributed Electricity Generation , http://www.cbo.gov/showdoc.cfm?index=4552andsequence=2.
Note : The url provided above returned invalid results.
Visit the homepage at
http://www.cbo.gov/Edison Electric Institute. (1988). EEI Pocketbook of Electric Utility Industry Statistics . Washington, DC: Edison Electric Institute.
Edison Electric Institute. (1997). EEI Pocketbook of Electric Utility Industry Statistics . Washington, DC: Edison Electric Institute.
Edison Electric Institute. (1988). Statistical Yearbook of the Electric Utility Industry/1987 Washington, DC: Edison Electric Institute.
Electric Light and Power . Various issues with statistics on heat rates (thermal efficiency) of steam power plants.
Electric Power Supply Association. (2005), "Competitive Power Supply Industry Facts," http://www.epsa.org/Competition/quick_facts_mp.cfm .
Electric Utility Consultants. (2004). The Installed Base of U.S. Distributed Generation . Greenwood, CO: EUC Publishing.
Fallek, Mark. (2004). "Distributed Generation: Electric Utility Perspective," http://uschpa.admgt.com/Rdmap03Fallek.pdf .
Friedman, S. Julio and Thomas Homer-Dixon. (2004). "Out of the Energy Box." Foreign Affairs 83(6) (November/December): 71-82.
Garman, David. (2005). "Diversification of Power Generation." Hearing Before the House Committee on Energy and Commerce (March 8). Washington, DC: Government Printing Services.
Halverson, Matthew. (2002). "Downturn in Demand Gives Contractors Time to get in the DG Game." ECandM (February), http://www.ecmweb.com/mag/electric_downturn_demand_gives/ .
Herzog, Antonio, Timothy Lipman, and Jennifer Edwards. (2001). "Renewable Energy: A Viable Choice," Environment (December): 1-34.
Hinrichs, Doug, Susan Conbere, and Mike Lobash. (2002). "Taking Control of Power Supplies." In Building Operating Management (July), http://www.findarticles.com/p/articles/mi_qa3922/is_200207/ai_n9110155 .
Hirsh, Richard F. (1989). Technology and Transformation in the American Electric Utility Industry . New York: Cambridge University Press.
Hirsh, Richard F. and Adam H. Serchuk. (1996). "Momentum Shifts in the American Electric Utility System: Catastrophic Change—Or No Change at All?" Technology and Culture 37: 280-311.
Hirsh, Richard F. (1999). Power Loss: The Origins of Deregulation and Restructuring in the American Electric Utility System . Cambridge, MA: MIT Press.
Hughes, Thomas P. (1983). Networks of Power: Electrification in Western Society, 1880-1930 . Baltimore: Johns Hopkins University Press.
Hughes, Thomas P. (1987). "The Evolution of Large Technological Systems." In Wiebe E. Bijker, Hughes and Trevor J. Pinch (Eds.) The Social Construction of Technological Systems: New Directions in the Sociology and History of Technology. Cambridge, MA: MIT Press.
Hughes, Thomas P. (1989). American Genesis: A Century of Invention and Technological Enthusiasm 1870-1970 . New York: Penguin Books.
Insull, Samuel. (1915). Central-Station Electric Service: Its Commercial Development and Economic Significance as Set Forth in the Public Addresses (1897-1914) of Samuel Insull . William Eugene Keily, (Eds.). Chicago: Privately Printed.
International Energy Agency. (2002). Distributed Generation in Liberalized Electricity Markets . Paris: International Energy Agency.
Kendrick, John W. (1961). Productivity Trends in the United States . Princeton, NJ: Princeton University Press.
Kendrick, John W. (1973). Postwar Productivity Trends in the United States, 1948-1969 . New York: Columbia University Press.
Kleinbach, Paul and Henry Hinrichs. (2002). Energy: Its Use and the Environment . New York: Harcourt College Publishers.
Landsberg, H. H. and S. H. Schurr. (1968). Energy in the United States: Sources, Uses, and Policy Issues . New York: Random House.
Lin, Jackie. (2004). "Power Outage Hits Industrial Park Hard." Taipei Times (April 11), p. 10.
Liss, William E. (1999). GRI Technical Paper: Natural Gas Power Systems for the Distributed Generation Market. New Orleans: Power-Gen International.
Lovins, Amory et al. (2002). Small is Profitable: The Hidden Benefits of Making Electrical Resources the Right Size . Snowmass, CO: Rocky Mountain Institute.
McCraw, Thomas K. (1984). Prophets of Regulation: Charles Francis Adams, Louis D. Brandeis, James M. Landis, Alfred E. Kahn . Cambridge, MA: Belknap Press of Harvard University Press.
Melosi, Martin V. (2000). T he Sanitary City: Urban Infrastructure in America from Colonial Times to the Present . Baltimore, MD: Johns Hopkins University Press.
Meyer, Niels I. (2003). "Distributed Generation and the Problematic Deregulation of Energy Markets in Europe." International Journal of Sustainable Energy 23(4): 217-221.
Noble, David F. (1999). "Social Choice in Machine Design: The Case of Automatically Controlled Machine Tools." In Donald MacKenzie and Judy Wajcman (Eds.), The Social Shaping of Technology . New York: Open University Press.
Ostergaard, Poul Alberg. (2003). "Heat Savings in Energy Systems With Substantial Distributed Generation." International Journal of Sustainable Energy 23(4): 169-176.
Pepermans, G. et al. (2003). "Distributed Generation: Definition, Benefits, and Issues." Energy Policy (August): 21-29.
Rendon, James. (2003). "In Search of Savings, Companies Turn to the Sun." New York Times (October 12): B12.
Rigby, Peter. (2003). "Deregulation's dysfunctional markets strike back; The blackout of '03 intensifies political and regulatory risk for U.S. transmission." Platts Energy Business and Technology 5(8): 28-35.
Sheer, Hermann. (2001). A Solar Manifesto: The Need for a Total Energy Supply and How to Achieve It . New York: Earthscan Publications.
Silberglitt, Richard, Emile Ettedgui, and Anders Hove. (2002). "Strengthening the Grid: Effect of High-Temperature Superconducting Power Technologies on Reliability, Power Transfer Capacity, and Energy Use," at http://www.rand.org/publications/MR/MR1531/.
Smith, Rebecca. (2004). "Not Just Tilting Anymore." Wall Street Journal (October 14), C1.
Stigler, George J. (1971). "The Theory of Economic Regulation." Bell Journal of Economics and Management Science 2 (Spring): 3-21.
U.S. Department of Energy, Energy Information Administration. (2000). Modeling Distributed Electricity Generation in the NEMS Buildings Models . Washington, DC: Department of Energy.
U.S. Department of Energy, Energy Information Administration. (2003) Form EIA-860 Database, Annual Electric Generator Report, at http://www.eia.doe.gov/cneaf/electricity/page/eia860.html .
U.S. Department of Energy, Energy Information Administration, Annual Energy Review 2003 (2004), at http://www.eia.doe.gov/emeu/aer/txt/ptb1306.html
U.S. Department of Energy. (2005a). Energy Information Administration Statistics, at http://www.eia.doe.gov/emeu/mer/pdf/pages/sec9_14.pdf .
U.S. Department of Energy. (2005b). "Electric Power Annual 2003," at http://www.eia.doe.gov/cneaf/electricity/epa/epa.pdf .
Wiser, Ryan and Ole Langniss. (2001). "The Renewables Portfolio Standard in Texas: An Early Assessment." Lawrence Berkeley National Laboratories Report LBNL-49107.
Yacobucci, Brent D. and Aimee E. Curtright. (2004). "A Hydrogen Economy and Fuel Cells: An Overview." CRS Report for Congress (January 14). Library of Congress: Congressional Research Service.
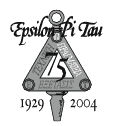
