JOTS v38n1 - Voices from the Past: Messages for a STEM Future
Voices from the Past: Messages for a STEM Future
Todd R. Kelley
ABSTRACT
The current emphasis in K-12 education on science, technology, engineering, and mathematics (STEM) ( Douglas, Iversen, & Kalyandurg, 2004 ; Sanders, 2009 ) creates many ways to partner engineering education with these fields. Therefore, it is appropriate to examine the commonalities these fields have with engineering education. Though much of the science education and mathematics education history is understood, technology education’s history is not common knowledge, and as a result misconceptions abound ( Daugherty & Wicklein, 1993 Wicklein, 2008 ). Technology education’s longstanding history in problem- and project-based learning, design- and engineering-related pedagogical approach is over a century old and grounded in theories of Comenius, Rousseau, Pestalozzi, Froebel, Herbart, Sheldon, and Dewey ( Dewey, 1915 ; Foster, 1995 , 1997 ; Herschbach, 2009 ; Kirkwood, 1994 ). The brief review of technology education’s history will reveal an almost eerie parallel to the current engineering education and STEM education movements.
Key Words: technology education, K-12 STEM education, project-based Instruction, design-based instruction
Introduction
Although other authors have visited various historical milestones in technology education origins such as manual arts, manual training, industrial arts, industrial technology ( Bonser, 1926 ; Foster, 1995 , 1997 ; Herschbach, 2009 ; Scott & Sarkees-Wircenski, 2004 ), this article will highlight some less explored milestones within technical education that provide opportunity for reflection on the current position held by technology education regarding the STEM paradigm. Some milestones featured here cross paths with early American engineering programs that emphasized project-based instruction while other milestones promote design-based instruction within technology education and general K-12 education. Finally, the focus of this historical journey will conclude with recent milestones that integrate core subjects with technology education. Recent approaches to K-12 STEM education appear to mix of these various pedagogical approaches to integrate the four subjects (science, technology, engineering, and mathematics); thus, this provides the rationale to revisit these voices of the past to speculate what these milestones might indicate for the success of a the T in STEM. Figure 1 illustrates both the milestones that are featured in this article and a general progression that led to the current STEM movement. Although many technology education milestones are not featured in this article, those selected here provide insight not only for technology education but also for engineering education, the two often-neglected members of the greater STEM community.
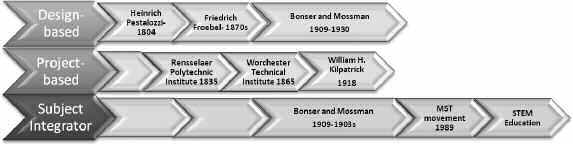
Early Design-Based Instruction
American’s early educational movement in the field better known today as technology education was heavily influenced by European education pioneers ( Herschbach, 2009 ). The father of the modern kindergarten movement, Friedrich Froebel, first studied under Johann Heinrich Pestalozzi. Both educational reformers believed heavily in educating children in a full range of real-life activities and using a hands-on approach to teaching. Pestalozzi focused on the object lesson, that is, using objects for concrete observations, which was later brought to the United States by Edward Austin Sheldon ( Foster, 1995 ). Pestalozzi began this work with orphans in Switzerland in 1804. Another pedagogical hands-on approach used by Pestalozzi was combining sketch work as a way to teach children how to learn and write letters from the alphabet. Froebel studied Pestalozzi’s methodologies and developed his own theoretical framework on the three realms, which included forms of nature (or life), forms of knowledge (or science), and forms of beauty (or art). These realms were introduced to children via short sessions of guided play with building blocks, mosaic toys, and traditional crafts. Children were taught to look for the mathematical equations in these objects.
The ultimate lesson of kindergarten was straightforward: the forms of the world, mathematics and art are equivalent and interchangeable. A chair made of eight cubes might become the number eight, then a pinwheel design, then a bed and so on. ( Brosterman, 1997 , p. 111)
Frobel actually marketed children’s building kits (e.g., manufactured by E. Steiger & Company and A. N. Myers & Company) in the 1860s-1870s as a way to study design and geometry. These kits included stick and cork geometric form building kits, carefully proportioned maple wood building blocks, colored cardboard triangles, and even child-size grid tables as a work surface to guide geometric pattern construction ( Brosterman, 1997 ).
Famous American architect, Frank Lloyd Wright was given these Frobel toys as a child. He credited his success in architecture with his early childhood experiences using the grid table, cardboard shapes, and the maple wood blocks. He believed the Frobel gifts were foundational to developing his abilities to design later in life ( Brosterman, 1997 ; Coleman, 2008 ). As engineering education and other STEM efforts seek to teach young learners about design and engineering, these STEM stakeholders should consider revising Frobel’s methodologies as tools to teach design. Consider recent engineering education efforts to teach children about engineering using toys such as LEGO® building blocks ( Capozzoli & Rogers, 1996 ; Connolly, Wendell, Wright, Jarvin, & Rogers, 2010 ; Erwin, Cyr, & Rogers, 2000 ). Frobel is just one of many unlikely educational pioneers who influenced the field of technology education; he is considered an unlikely influencer because his contribution is neither technical nor vocational, but it is design and aesthetically focused.
Technology Education for All
Other pioneers in the history of technology education also focused on the design and aesthetics of the study of technology. Frederic Bonser and Lois Coffey Mossman had a profound influence on the early beginnings of technology education, and much of their work focused on the elementary grades. Bonser and Mossman believe that all children should receive manual training and industrial education, and the purpose was social reform, not vocational education. Mossman is credited for being the first person to use the term Industrial Arts by 1909, when she proposed the combination of manual training, drawing, and home economics in elementary and middle grades ( Foster, 1995 ). In addition to aligning the school’s practical work with the traditional curriculum, Mossman emphasized the need for students to design their own projects. When learning about clothing, some students designed and made their own shirtwaists; when learning about shelter, students planned and drew houses (“On the ground floor,” Coffey-Mossman, 1907, p. 123, in Foster, 1995 ). Although Bonser and Mossman provided many publications outlining their educational philosophies related to industrial arts ( Bonser, 1911 , 1926 , 1932 ; Bonser & Mossman, 1923 ; Mossman, 1924 , 1929 , 1938 ) much of their conception of industrial arts was lost when implemented in the classroom ( Foster, 1995 ). This example should serve as a warning to STEM education leaders and educational policy makers that theory (educational philosophy) and practice (in-service teacher pedagogy) do not always mesh. If researchers of STEM fail to thoroughly investigate the complexities surrounding teacher practices that integrate STEM, the recent efforts to infuse STEM education into the classroom will be void.
Project-based Learning in Technology Education and Engineering
The early roots of technology education are closely intertwined with the development of the American engineering schools. Van Rensselaer, founder of Rensselaer Polytechnic Institute (RPI) in Troy, New York, along with Amos Eaton are credited for creating a university founded on teaching of the sciences through practical application, such as applying mechanical philosophy to the machinery of steamboats, mills, and factories. The practical applications of science at Rensselaer also included surveying land; calculating water pressures in locks, aqueducts, and dams; and designing and planting experimental gardens. These examples of pedagogical approaches to the sciences led to the founding of a department of Mathematical Arts at Rensselaer in 1835 “for the purpose of giving instruction in Engineering and Technology” ( Bennett, 1926 , p. 353). This is the first example of an American civil engineering school in the United States; four students graduated from RPI with civil engineering degrees in 1835, and before that time civil engineers were imported from France to build U.S. canals and bridges ( Bennett, 1926 ).
Another important early American school of mechanical engineering that combined the theory and practice of engineering through scientific reasoning and shop experience was the Worcester Technical Institute in Worcester, Massachusetts, which was founded in 1865. Several donors, including John Boynton and Ichabod Washburn, two prominent Worcester industrialists, helped to make the Worchester Technical Institute a reality. It was Washburn, however, who added a unique element to the school curriculum; he wanted to teach vocational skills while Boynton wanted to teach science. A machine shop was donated to the institute to provide practical application to the science instruction. “Thus it came about that a new type of mechanical engineering course was made possible—a course which combined experience in a shop . . . and a theoretical course in applied science and engineering” ( Bennett, 1926 , p. 360). Bennett (1926) wrote that the shop was not for manual or industrial training but for educational purposes; the machine shop was considered as educational as a laboratory is to science. The work done in the machine shop was to be a substitute for an apprenticeship while the students simultaneously took mathematics, science, and engineering courses ( Bennett, 1926 ). The news spread about the success of this educational approach and other universities around the country began to introduce shop work into their engineering programs ( Scott & Sarkees-Wircenski, 2004 ). Although there were proponents of the practical application of mathematics, science, and engineering through hands-on manual education, many people during the decade from 1880-1890 had great opposition to manual training in K-12 classrooms, and as a result, the gap between academic subjects and hands-on activities began to widen ( Bennett, 1926 ).
If one fast-forwards from Rensselaer’s 1835 era of practical application of engineering education to engineering education in the 21st century, one can make an eerie parallel. Authors Dym, Agogino, Eris, Frey, and Leifer (2005) explored the complexity of engineering design thinking and how design is best taught in engineering. Interestingly, these authors cited project-based learning (PBL) as the most favorable pedagogical approach for teaching design within engineering education. Moreover, Dym et al. (2005) suggested that the best context for PBL is first-year engineering cornerstone courses because it delivers a key element understood in cognitive science transfer of learning which “allows students to apply what was learned in new situations and to learn related information more quickly” ( Bransford, Brown, & Cocking, 1999 , p. 17). Dym et al. (2005) also indicated that PBL motivates students to learn upper level engineering sciences and helps with student retention in engineering schools. Brophy, Klein, Portsmore, and Rogers (2008) provided a comprehensive overview of engineering education curriculums and research efforts in P-12 classrooms within the United States, and once again PBL and other hands-on design-based instruction dominate the highlighted pedagogical approaches. The original founder of the project-based learning (PBL) concept was William Heard Kilpatrick. In September of 1918, Kilpatrick presented this theory of learning in an essay titled The Project Method , which emphasized "purposeful activity" to engage students in the learning process as they worked on a variety of projects. At the time, this new pedagogical approach was so popular that over 60,000 copies of the essay were published in pamphlet form ( http://www.answers.com/topic/william-hkilpatrick ); later this information was published in book form entitled Foundations of Method ( Kilpatrick, 1925 ). It was widely accepted in technology education (Industrial Arts) during that era.
It was also an attractive idea that activity could serve as the center for correlating subject matter from a number of subject areas, such as English, math, science, and industrial arts. In this way, students could learn how to apply formal knowledge to the immediate concerns of their daily lives. ( Herschbach, 2009 , p. 33-34)
The current proposed approaches to K-12 STEM education and design- and project-based engineering education have origins in the works of both Kilpatrick and Rensselaer and the ideas of the Worcester Technical Institute. Some of the concepts of Rensselaer’s engineering school of 1835 live on in today’s modern engineering programs. Purdue’s Neil Armstrong Hall of Engineering was completed in 2008. This new faculty includes multiple material testing and fabricating laboratories to provide hands-on design experiences. According to the Purdue University website, “Industry is demanding engineers who have traditional technical expertise along with design and building experience, often on industrial scale projects, and who can work in diverse teams” (see https://engineering.purdue.edu/Engr/AboutUs/Facilities/ArmstrongHall/Features/ ). These are several of examples of the history of technology education and engineering that illustrate that both fields are returning to their pedagogical roots by providing practical applications of design and engineering instruction. Although both fields often promote these methods as new innovations, the reality is that these approaches to education are well over a century old.
Technology Education is a Subject Integrator
There are many examples of subject integration within the history of technology education that can provide lessons to the STEM community. It is likely that current technology educators would be shocked to find so many examples of subject integration within the early forms of technology education (manual arts, manual training, and industrial arts). “Current efforts in technology education to integrate math, science, and technology also have an extensive heritage, although much of that heritage has either been neglected or ignored” ( Pannabecker, 2004 , p. 78). The industrial arts pioneer, Lois Coffey Mossman repeatedly emphasized in her writings that the integration of school subjects could be achieved through practical classroom activities. For example, she discussed the use of poems in a lesson in agriculture. Mossman emphasized the need to connect the study with arithmetic, geometry, reading, art, geography, nature study, physics, and botany (Coffey as cited in Foster, 1995 ). Although the roots of technology education as a subject integrator grow deep, what has occurred in the classroom provides little solid evidence of a widespread effort to use technology education as a vehicle to integrate subjects. Sanders (2009) indicated that technology education teachers like to boast about teaching science and mathematics but often fail to do so in practice. However, in the late 1990s, there were some efforts to reform the curriculum to conscientiously integrate math, science, and technology.
The MST Approach
One example of an educational approach to include technology education through a multi-disciplinary approach to improve mathematics and science was the MST movement. Many educational leaders in the early 1990s recognized the need to improve American students’ scores in science and mathematics. Documentation of the status of student achievement in math and science during this time can be found in reports such as Everybody Counts: A Report to the Nation on the Future of Mathematics Education ( National Research Council, 1989 ) and Project 2061: Science for All Americans ( American Association for Advancement of Science, 1989 ). One approach considered to improve scores in these core subjects was to include technology education though design-based instruction as a way to provide a context for learning math and science. Many technology educators believed the MST approach as an outstanding opportunity for the field to be included with core subjects to provide exposure of technology education to all learners. Laporte and Sanders (1993) believed that the MST approach would improve the status of technology education; however, there were other leaders who wondered if technology education would become a stepchild in the ‘marriage of math and science’ ( Foster, 1994 ; Gloeckner, 1991 ). Daugherty and Wicklein (1993) probed deeper to determine math, science, and technology teachers’ perceptions of technology education. Their findings revealed that some misconceptions and poor perceptions of technology educators and the field technology education existed. Possible reasons for misconceptions about technology education were stated previously based upon the general public’s exposure to industrial arts. Hansen (1995) in a study on teacher socialization within technology education provided another possible explanation. Hansen’s study found that technology education teachers tend to congregate with peers within the field of technology education and as a result fail to connect with the greater learning community. Therefore, the technology educators have limited impact on how teachers in other content areas view and perceive the technology education field and local technology programs. This discovery within technology education should be considered as teacher professional development brings together STEM teachers to collaborate to integrate STEM disciplines within the classroom.
One of the best examples of the MST approach was the New York State Technology Education Network (NYSTEN), a project funded by the National Science Foundation. The goals of NYSTEN were to provide contemporary approaches of technology education, conduct educational research on technology education classrooms, and provide professional development opportunities and leadership opportunities to technology educators across New York State ( Burghardt & Hacker, 2002 ). The MST movement began to die out when federal dollars ran out, such as Goals 2000 funding, and the MST approach lost significant momentum. Efforts such as the MST standards document for New York state were not implemented as originally designed. High stakes testing also played a role in ending the MST movement in New York. The New York State Education Department website states the following:
Through the foresight of many, the standard for technology and technology education programs was linked to mathematics and science. Illustrating the interconnectedness of these three subjects the Mathematics, Science, Technology (MST) Learning Standards has created a dynamic force for demonstrating student knowledge. While mathematics and science have had a long history in education, technology education is a relatively new subject with less stature and acceptance. Added to this the testing pressures placed on mathematics and science education, technology education has been overlooked as a tool for improving student achievement. ( New York State Education Department, 2006 , p. 4)
These examples reiterate the concerns of Gloeckner (1991) and Foster (1994) , and others leaders who believe that technology education will never be considered a viable school subject required for study by all students. The greater STEM community must learn from these past events in order to ensure that all STEM stakeholders are well understood. Unfortunately, the leaders from the MST movement failed to fully investigate the complexities of the community of MST teachers; therefore, there was little effort to overcome negative stereotypes or misconceptions of the three subject domains that often stifled building strong teacher partnerships.
Communicating a Clear Mission
Technology education is a very broad term, and that large scope may contribute to its the many misperceptions. Furthermore, there are multiple purposes for teaching technology education in secondary education that have caused great confusion about the purpose of technology education. Although many technology educators today would contend that technology education is important for all students ( ITEA, 1996 ) and that this has been the general mission of technology education since the early 1900s ( Foster, 1997 ); however, there are other educators who focus on technology education as a career pathway. Foster and Wright (1996) document the tension in the 1970s between proponents of industrial arts education for all students versus vocational education. Both purposes of technical education existed and completion for students and funds split resources as well as continued to blur the mission of technology education. Today, divisions still exist in professional teacher education associations with the simultaneous existence of the Technology Education Division (TED) within the Association for Career and Technical Education (ACTE) and International Technology and Engineering Education Association (ITEEA) ( Hill, 2006 ). There are many authors who have written about the lack of uniformity within technology education ( Petrina, 1993 ; Wicklein, 2006 ; Wright, 1992 ).
As the engineering education community continues to approach the K-12 arena, it will be critical that engineering educators examine this page from technology education’s history. Engineering education must define the main purpose of its role in K-12 education. Is the purpose to improve STEM learning for all children or is the purpose to provide a career pipeline to engineering? Currently, the engineering education literature suggests that both missions for K-12 engineering education exist ( Brophy et al., 2009 ; Douglas et al., 2004 ; National Academy of Engineering, 2004 ; Committee on Prospering in the Global Economy of the 21st Century, 2007 ). Moreover, the funding opportunities that exist in STEM education can cloud the mission of any educational venture. Currently there are over 45 NSF programs that include STEM somewhere in the RFP solicitation ( www.nsf.gov , date accessed 5/30/12). Back in 2009, a $100 million of the $787 billion stimulus package was designated for the National Science Foundation ( Riley, 2009 ). In technology education, this “clouding the mission” occurred as early as the late 1800s. Lewis (1996) cited Woodward (1894) as a proponent of manual arts for all children’s general education who compromised his ideals of a liberal education of manual arts to an approach to manual training as trade training to acquire needed funds from the Smith-Hughes Act of 1917. Current concerns within the technology education community exist about aligning with engineering education and the greater STEM movement—this could cloud the mission of technology education. “If engineering groups start exercising too much influence on technology education, our field risks a greater association with engineering’s vocational or professional orientation, its perceived loyalty to business, and its traditional political alliances” ( Pannabecker, 2004 , pp. 79-80). Another concern for technology educators is that “by focusing heavily on pre-engineering, technology education also is ‘vocationalized’ to the extent that it is limited primarily to prepare youth to enter into a specific occupational field” ( Herschbach, 2009 , p. 240). It is quite possible that members of technology education express these concerns because engineering education has not communicated its intentions or purpose for K-12 engineering education.
Salinger (2005) has suggested that the study of K-12 engineering should not be vocational but it should be taught as a way of thinking. In his paper The Engineering of Technology Education , he challenges technology educators to use the process outlined in Understanding by Design ( Wiggins & McTighe, 2005 ) to help the field of technology education establish its mission regarding teaching engineering within technology education. Salinger (2005) suggested technology educators ask themselves the question, “What is the goal?” In other words, they should identify what the purpose is to infuse engineering concept into technology education. The author of this article believes the same question can be applied to engineering education regarding its role in K-12 education: What is the goal?
Conclusion
The overarching purpose of this article was to share some commonalities between technology education and engineering education and strong, almost eerie parallels of technology education history with engineering education and the current STEM education movement. Salinger (2005) provided this challenge to the field of technology education:
The technology education profession has worked hard on the issue of the content of its discipline and on how to be educated to teach it ( ITEA, 2000/2002 ); but perhaps needs to think more strategically about other dimensions like where can it get support? (p. 3)
The author of this article would like to suggest that T and the E should work harder to provide support for one another. Of all the STEM stakeholders who sit at the “STEM table,” members of the technology and engineering fields are best positioned to sit the closest; as a result their contribution to K-12 STEM education will be strengthened. Consider the words of engineering educators Haghighi, Smith, Olds, Fortenberry, and Bond (2008) as they spoke regarding engineering education’s future: “We must form win-win partnerships not only in areas such as cognitive science but also in the interdisciplinary context of the larger engineering college as well as the K-12 community and community colleges” (p. 120).
Taking a page from Frans Johansson’s (2004) best-selling book: Medici Effect: Groundbreaking Innovation at the Intersection of Ideas, Concepts, & Cultures , the author of this article suggests that technology education and engineering education strengthen their positions within STEM by locating the key intersections within these fields of study. If fields suffer from a lack of a clear mission, partnering to explore common intersections would be prudent. Possibly one of the greatest opportunities to begin to define a clear mission is to simultaneously establish a clear research agenda. What are the intersections between a technology education research agenda and an engineering education research agenda? This author believes that both fields must discover through research the benefits that exist in teaching engineering in K-12 classrooms. Does teaching in the context of engineering design improve students’ STEM learning? These are just a few questions that should be asked by researchers within technology education and engineering education. Unfortunately with current state’s budget constraints in K-12 education, the T and the E are in possible jeopardy of funding cuts; some cuts have already occurred. It is now time to conduct rigorous research that speaks loudly to policy makers—a strong partnership between the T and the E can begin to build a clear research agenda.
Dr. Todd R. Kelley is an assistant professor in the College of Technology (and P-12 STEM Researcher) at Purdue University, West Lafayette, Indiana. He is a member of Phi Chapter of Epsilon Pi Tau.
References
American Association for Advancement of Science. (1989). Project 2061: Science for all Americans . Washington, DC: Author.
Bennett, C. A. (1926). History of manual and industrial education up to 1870 . Peoria: Bennett.
Bonser, F. G. (1911). Fundamental values in industrial education. Teachers College Bulletin III (November 18). New York: Teacher’s College, Columbia University.
Bonser, F. G. (1926). A history of industrial arts in Teachers College, to May, 1926 . [Unpublished manuscript, Milbank Memorial Library, Teachers, College, Columbia University, New York.]
Bonser, F. G. (1932). Bibliography. In life needs and education (pp. 271-288). New York: Teachers College, Columbia University.
Bonser, F. G., & Mossman, L. C. (1923). Industrial arts for elementary schools . New York: Macmillan.
Bransford, J. D., Brown, A. L., & Cocking, R. R. (1999). (Eds.). How people learn: Brain, mind, experience, and school , Washington, DC: National Academy Press.
Brophy, S., Kelin, S., Portsmore, M., & Rogers, C. (2008). Advancing engineering education in P-12 classrooms. Journal of Engineering Education . 369-382.
Brosterman, N. (1997). Child’s play. Art in America, 85 (4), 108-111, 130.
Burghardt, D., & Hacker, M. (2002). Large scale enhancement projects focusing on technology education. Journal of Industrial Technology Education, 39 (3), 88-103.
Capozzoli, P., & C. Rogers. 1996. LEGOs and aeronautics in kindergarten through college In Proceedings of the Advanced Measurement and Ground Testing Technology Conference . New Orleans, LA.
Coleman, D. (2008). Long before Legos, wood was nice and did suffice. The New York Times, 11.
Connolly, K. G., Wendell, K. B., Wright, C. G., Jarvin, L., & Rogers, C. (2010). Comparing children's simple machines learning in LEGO(TM) engineering-design-based and non-LEGO engineering-design-based science environments. Paper presented at the Annual International Conference of the National Association for Research in Science Teaching (NARST), Philadelphia, PA.
Committee on Prospering in the Global Economy of the 21st Century (2007). Rising above the gathering storm: Energizing and employing America for a brighter economic future. National Academy of Science. Washington, DC: National Academies Press.
Daugherty, M.K., & Wicklein, R.C. (1993). Mathematics, science, and technology teachers’ perceptions of technology education. Journal of Technology Education, 4 (2), 28-43 .
Dewey, J. (1915, May 15). Education vs. trade-training. New Republic , pp. 40-43.
Douglas, J., Iversen, E., & Kalyandurg, C. (2004). Engineering in the K-12 classroom: An analysis of current practices and guidelines for the future. A production of the ASEE Engineering K12 Center.
Dym, C., Agogino, A., Eris,O., Frey, D., & Leifer, L (2005). Engineering design thinking, teaching, and learning. Journal of Engineering Education, 95 (1), 103-120.
Erwin, B., Cyr, M., & Rogers, C. 2000. LEGO engineer and ROBOLAB: Teaching engineering with LabVIEW from kindergarten to graduate school. International Journal of Engineering Education 16(3), 181–92.
Foster, P. (1994). Must we MST? Journal of Technology Education, 6 (1), 76-84 .
Foster, P. N. (1995). The founders of Industrial Arts in the U.S. Journal of Technology Education. 7 (1), 6-21 .
Foster, P. N. (1997). Lessons from history: industrial arts/ technology education as a case. Journal of Vocational and Technical Education , 13(2), 5-15 .
Foster, P. N., & Wright, M. D. (1996). Selected leaders’ perceptions of approaches to technology education. Journal of Technology Education, 7 (2), 13-27 .
Gloeckner, G. (1991). The integration of science, technology, and mathematics myth or dream? Journal of Technology Education, 2 (2) 1-7 .
Haghighi, K., Smith, K.A., Olds, B. M., Fortenberry, N., & Bond, S. (2008). The time is now: Are we ready for our role? Journal of Engineering Education, 97 (2), 119-121.
Hansen, R. E. (1995). Teacher socialization in technological education. Journal of Technology Education, 6 (2), 34-45 .
Herschbach, D. (2009). Technology education: Foundations and perspectives . Homewood, IL: American Technical Publishers, Inc.
Hill, R. B. (2006). New perspectives: Technology teacher education and engineering design. Journal of Industrial Teacher Education, 43 (3), 45-63 .
International Technology Education Association. [ITEA]. (1996). Technology for all Americans: A rationale and structure for the study of technology . Reston, VA: Author.
International Technology Education Association. [ITEA]. (2000/2002) Standards for technological literacy: Content for the study of technology . Reston, VA: Author.
Katehi, L., Pearson, G., & Feder, M. (Eds.). (2009). Engineering in K-12 education: Understanding the status and improving the prospectus . Washington, DC: National Academies Press.
Johansson, F. (2004). The Medici effect: Breakthrough insights at the intersection of ideas, concepts, & cultures . Cambridge, MA: Harvard Business School Press.
Kirkwood, J. J. (1994). Construction in elementary school technology education. In J. W. Wescott & R. H. Henak (Eds.). Construction in technology education (pp. 75-101). Peoria, IL: Glencoe.
Kilpatrick, W. H. (1918, September). The project method. Teachers College Record, 19 (4), p. 319–35.
Kilpatrick, W. H. (1925). Foundations of method . New York: Macmillan.
LaPorte, J., & Sanders, M. (1993). Integrating technology, science, and mathematics in the middle school. The Technology Teacher, 52 (6), 17-21.
Lewis, T. (1996). Accommodating border crossings. Journal of Industrial Teacher Education, 33 (2), 7-28 .
Mossman, L. C. (1924). Changing conceptions relative to the planning of lessons . New York: Teachers College, Columbia University.
Mossman, L. C. (1929). The content of the social studies in the elementary school. Teachers College Record, 30 (4), 322-333.
Mossman, L. C. (1938). The activity concept: An interpretation . New York: Macmillan.
National Academy of Engineering. (2004). The Engineer of 2020 . Washington, DC: The National Academy Press.
National Research Council (1989). Everybody counts: A report to the nation on the future of mathematics education . Washington, DC: National Academy Press.
New York State Education Department. (2006). NYS Technology Education Framework Initiative, p. 4. Retrieved September, 15, 2009. http://www.emsc.nysed.gov/cte/technology/initiative/home.html
Pannabecker, J. (2004). Technology education and history: Who’s driving? Journal of Technology Education, 16 (1), 72-83 .
Petrina, S. (1993). Diversity, not uniformity united, not standardized: A reaction to Wright’s “challenge to all technology educators.” Journal of Technology Education, 4 (2), 69-76 .
Riley, S. (2009, March, 10). Tech education will get some funding from stimulus effort. Investor’s Business Daily Inc .
Salinger, G. (2005). The engineering of technology education. Journal of Technology Studies, 31 (1), 2-6 .
Sanders, M. (2009) STEM, STEM education, STEMmania. The Technology Teacher, 68 (4). 20-26.
Scott, J. L., & Sarkees-Wircenski, M. (2004). Overview of career and technical education . Homewood, IL: American Technical.
Wicklein, R. C. (2006). Five good reason for engineering design as the focus for technology education. The Technology Teacher, 65 (7), 25-29.
Wiggins, G. P., & McTighe, J. (2005). Understanding by design . (2nd ed.). Alexandria, VA: Association for Supervision and Curriculum Development.
Woodward, C. (1894). New demands upon schools by the world’s industries . Proceedings of the International Congress of Education of the World’s Columbian Exposition, New York: National Education Association.
Wright, T. (1992). Building a defensible curriculum base. Journal of Technology Education, 3 (2), 62-66 .