JOTS v37n2 - Design, Operation, and Analysis of a Floating Water Fountain System Using Renewable Energy Technology
Design, Operation, and Analysis of a Floating Water Fountain System Using Renewable Energy Technology
Hans Chapman, Eduardo Gomez, Nilesh Joshi, and Sanjeev Adhikari
Abstract
Engineering and technological applications of renewable energy installations, such as photovoltaic (solar) energy, are making important contributions toward the development of environmentally friendly products and processes for a more sustainable future. This article presents the design, assembly, and operation of a solar powered floating fountain system for analysis of aeration in stagnant water. The goal was to increase the level of dissolved oxygen in a body of water by harnessing solar energy for submerged aeration. The system is composed of six solar panels, a kit of batteries, a linear current booster, pressurized water tank, two pumps, an air compressor, and a float. The design factors for dissolved oxygen (DO) measurements were determined from depth of water, time of the day, location of fountain, and status of fountain (on or off). A Split Plot design was used to investigate the performance of the fountain, based on the changes in levels of DO in the pond. Statistical analysis showed a 120% gain in DO concentration during a 20-day period with significant destratification of the pond. This applied research will be of interest to engineers and technologists in various areas, including environmental development, green construction, and aquatic and energy conservation.
Keywords: Renewable Energy Technology, Solar Powered Fountain, Aeration, Dissolved Oxygen.
Introduction
Photovoltaic (PV) renewable energy systems offer new alternatives for consumers and businesses as to how power can be provided. PV systems react to light by transforming part of the radiant energy into electricity. PV cells require no fuel to operate, produce no pollution, require little maintenance, and are modular. These attributes permit a wide range of solar - electric applications ( Markvart, 1999 ; Marshall & Dimova-Malinovska, 2002 ; Dunlap, 2010 ). Other advantages of PV systems include: unlimited input solar energy, reliable power output, flexibility in assembly, and easy installation ( Boyd, 1997 ; Butler & Sinton, 2004 ).
Pumps are useful devices for the operation of water fountains. Fountains are installed on water bodies, like ponds, streams, and lakes, to prevent destratification of the water ( Michaud & Noel, 1991 ; Lynch & Commer, 1994 ). Water pumping is one of the most competitive areas for PV power. PV pumping systems pump most water during the sunniest, hottest days of summers. PV pumping systems have, as a minimum, a PV array, a motor, and a pump. In addition to water pumping, PV systems can also be useful for aeration of water bodies.
Natural stream purification processes require adequate dissolved oxygen levels in order to provide for aerobic life forms. When pollution enters a body of water, plant and algae die and sink to the bottom, resulting in an overload of organic sludge. A lower form of life in lakes and ponds die and this debris eventually rots. Oxygen solubility has been shown to increase with decreasing temperature, salinity and pressure ( Wieland & Kühl, 2006 ). When dissolved oxygen (DO) is at saturation level, the number of oxygen molecules leaving the water surface equals the number entering (no net movement) ( Michaud & Noel, 1991 ). Below DO saturation, there is a net movement of oxygen from atmosphere to water. The greater the difference between the oxygen pressures in the water and the atmosphere, the larger the movement of oxygen from the atmosphere to the water.
System Design
The solar powered water fountain (SPOWF) was designed to enhance dissolved oxygen levels in a test pond at Innovation Park, Tallahassee, Florida. The three primary components for producing electricity using solar power are: solar panels, a charge controller, and batteries. Solar panels charge the batteries, and the charge regulator or linear current booster (LCB) ensures proper charging of the battery. The system was designed in two main parts (the fountain and the aerator). The water fountain adds oxygen to the water body by exposing the water to the external air. The aerator provides air bubbles into the body of water by using an air supply line from an air compressor. A different electrical circuit was designed for each part. The required solar panels and batteries were placed onshore and the loads were located offshore. The loads of the fountain include a submersible pump, a surface pump, and the air compressor.
Design of the Fountain
The fountain design consists of (i) solar array, (ii) deep cycle batteries, (iii) pumps, and (iv) pressure tank. The loads for the fountain are shown in Table 1.
Description | Volts | Amps | Watts | Amp –hour /day |
---|---|---|---|---|
Submersible pump | 24 | 4 | 96 | 48 |
Surface pump | 24 | 6 | 144 | 72 |
The number of solar modules in parallel required was 3. It was also determined that 2 modules in series were required for the 24 Volts battery, making a total of 6 batteries.
Deep-cycle Batteries
The capacity of the deep-cycle batteries used for a total of 432 Amp-hours (assuming 3 days in the week without sun and a correction factor of 1.2) was determined to be 4 batteries in series.
Pumps and Pressure Tank
Two pumps were chosen:
- A positive displacement 3-chamber diaphragm submersible pump with a total vertical lift of 70 meters, a flow rate of 8.6 x 10-4 m 3 /s, and a maximum pressure of 6.9 x 105 N/m 2 .
- A surface pump with a vertical lift of 9.1 meters, a flow rate of 3.3 x 10-5 m 3 /s, and a maximum pressure of 3.1 x 105 N/m 2 .
Figure 1 is a schematic diagram of the fountain showing how the panels, batteries, actuator, and pumps are connected. Considering the pressure and flow rate of the pumps, a 0.13 m 3 tank was chosen. Additionally, a "cut-in 2.1 x 105 N/m 2 / cut-out 3.4 x 105 N/m 2 " pressure switch and a pressure gauge were attached to the tank.
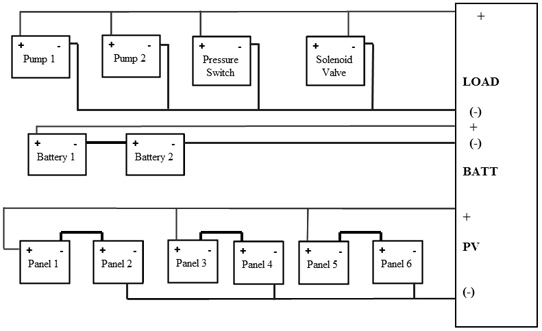
Design of the aerator
The aerator is comprised of one item each from the following: 7.2 Amps solar panel, 6- Amp air compressor, 6.1 m #12 AWG wire, 15 Amps charge regulator, 12 Volts battery, and a plastic case. A 0.13 m diameter, 1.68 m long PVC pipe was chosen, and a 90° elbow was glued at the top of the pipe to transport the air bubbles from the bottom to the surface of the lake. Four radial holes were drilled six inches from the end of the pipe to hold the air stones in place, using plastic fittings. The air stones were interconnected to a 9.5 x 10 -3 m flexible hose. The hose was connected to the air compressor located on top of the fountain as shown in Figure 2. The PVC pipe was then strapped with two 0.91 m x 0.04 m aluminum flat bars. One side was welded to the SPOWF aluminum structure, and the other side bolted to the pipe as showed in Figure 2.
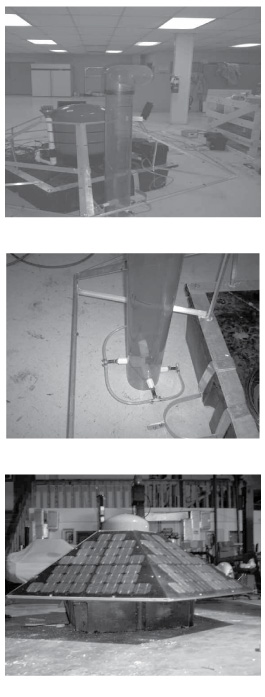
System Operation
When the SPOWF system is turned on, both pumps constantly pump water into the tank through the one-way valve. As the water flows into the tank, the pressure in the tank increases steadily to 3.44 x 10 5 N/m 2 . At this critical pressure, water is jetted of the fountain through the nozzles. The flow stops when the pressure drops below 2.1 x 10 5 N/m 2 , and the pressure switch shuts off to rebuild the pressure in the tank. This sequence is continued as long as there is enough current flow to power the loads ( Braimah, 2004 ; Gomez, 2006 ).
The purpose of running both the fountain and aerator together was to maximize the daily water circulation. The voltage output of the PV panels is often too low to run the pumps under these conditions. To offset this limitation, it was necessary to operate an energy booster with the system. The linear current booster (LCB) works by enhancing the output current from two batteries, especially under low light conditions, cloudy days, and early morning or late evening.
System Analysis
Cause-and-effect diagram
A cause-and effect diagram was developed for the SPOWF system as shown in Figure 3. From this diagram, the factors were classified as design factors, factors held-constant, and nuisance factors. The design factors were: depth of the water, time of the day, location of the fountain, and the status of the fountain i.e., ON or OFF. The factors held-constant were variables that could modify the response, but for the purpose of this experiment, these factors were not of interest, so they were held at their specific level. Some of them were: filters, fountain, solar panels, and pumps. Nuisance factors, on the other hand may have a large effect that must be accounted for. The nuisance factors considered in this experiment were: barometric pressure, temperature of the water, cloudiness, and air temperature.
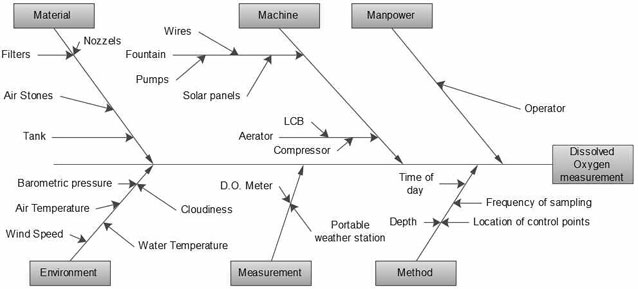
Dissolved oxygen measurement
The dissolved oxygen meter provided the reading of the concentration of dissolved oxygen as well as the depth and temperature of the water. Measurements were done every day at 7:00 AM and 1:00 PM during 20 consecutive days in October and November. Table 2 shows the data set after measurements were taken.
Day | Depth of water (meters) | Time of day (hours) | Location (meters) | Status of Fountain (On / Off) | Air Temp (°K) | Water Temp (°K) |
Cloudiness
(0 or 1 ) |
Wind speed
( m/s) |
DO
(mg/L) |
---|---|---|---|---|---|---|---|---|---|
1 | 0.3 | 13 | 1.52 | Off | 291 | 293 | 0 | 2.24 | 4.5 |
2 | 1.52 | 13 | 7.62 | Off | 291 | 291 | 1 | 0.45 | 4.7 |
3 | 0.3 | 7 | 7.62 | Off | 280 | 293 | 1 | 0 | 4.8 |
4 | 0.91 | 10 | 4.57 | Off | 291 | 292 | 0 | 1.34 | 4.6 |
5 | 1.52 | 7 | 1.52 | Off | 279 | 291 | 0 | 0.89 | 4.5 |
6 | 1.52 | 7 | 1.52 | On | 279 | 291 | 0 | 0.45 | 6.4 |
7 | 0.3 | 7 | 7.62 | On | 282 | 291 | 1 | 1.34 | 7.1 |
8 | 0.3 | 13 | 1.52 | On | 301 | 298 | 0 | 0.89 | 6.1 |
9 | 0.91 | 10 | 4.57 | On | 292 | 295 | 0 | 1.34 | 9.8 |
10 | 1.52 | 13 | 7.62 | On | 295 | 291 | 0 | 0.89 | 7.6 |
11 | 1.52 | 13 | 7.62 | On | 297 | 292 | 1 | 0.45 | 10 |
12 | 1.52 | 7 | 1.52 | On | 283 | 294 | 1 | 1.34 | 6.4 |
13 | 0.91 | 10 | 4.57 | On | 295 | 295 | 0 | 0.89 | 6.3 |
14 | 0.3 | 13 | 1.52 | On | 299 | 296 | 1 | 0.45 | 7.1 |
14 | 0.3 | 13 | 1.52 | On | 299 | 296 | 1 | 0.45 | 7.1 |
16 | 0.3 | 13 | 1.52 | Off | 300 | 302 | 1 | 1.34 | 4.6 |
17 | 0.91 | 10 | 4.57 | Off | 295 | 299 | 0 | 1.34 | 4.7 |
18 | 0.3 | 7 | 7.62 | Off | 285 | 299 | 1 | 1.79 | 4.6 |
19 | 1.52 | 7 | 1.52 | Off | 282 | 293 | 1 | 2.24 | 4.8 |
20 | 1.52 | 13 | 7.62 | Off | 295 | 296 | 1 | 2.24 | 4.5 |
Estimated general linear relation for final model
The final estimated model equation in terms of coded units with all the statistically significant terms was determined for two scenarios, i.e., “Fountain Off ” and “Fountain On” as indicated below:
Fountain OFF
DO = 4.63 - 0.5244 (Location) + 0.3569 (time of day) (7)
Fountain ON
DO = 6.0455 – 1.0238 (Location) + 0.7138 (time of day) (8)
Interactions between “fountain with location” and “fountain with time of day” are evident in the differing coefficient estimates for location and time of day for “fountain off ” vs. “fountain on”, respectively. A response surface analysis ( Simpson, Kowalsky, & Landman, 2004 ) was performed and the results are shown in Figure 4.
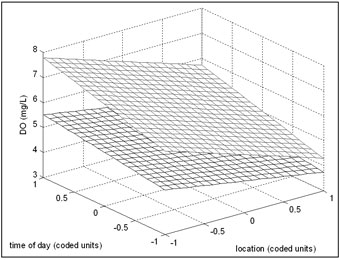
In order to accurately predict DO as a function of location and time of day, a transformation from natural to coded units ( Myers & Montgomery, 2002 ; Montgomery, 2005 ) should be made prior to applying the model as shown in Eqs. 7 and 8, respectively.
In general,
Coded units = (NU - [
Min
+
Max
]/2) / ([
Max
-
Min
]/2) (9)
Where, NU is the value in natural units
Min
is the (-1) value of the factor in natural units
Max
is the (+1) value of the factor in natural units.
Table 3 shows an example for calculating DO at a location, 3.7 m away from the fountain at 9:00 AM.
Natural Units | Coded Units | DO (mg / L) | |||
---|---|---|---|---|---|
Location | Time of Day | Location | Time of Day | ON | OFF |
3.7 m | 9:00 AM | -0.30 | -0.33 | 6.1147 | 4.66 |
Final model charts
The first datum for the fourth subplot was collected again, after 12 days, with the fountain in the OFF status. It was observed that the aeration effect due to the floating fountain had disappeared. Consequently, the measured DO levels were at the original values as shown in Figure 5. This new information is valuable because it can be inferred that the experiment was time independent.
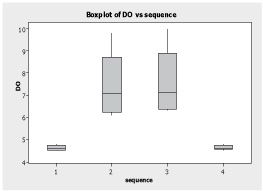
The interaction between the fountain and its location is shown in Figure 6. When the aeration system was in the OFF status, the DO concentration was observed to be 4.95 mg / L at 5 feet away from the fountain. At the same location when the aeration system was in the ON status, the value for DO was 8.8 mg / L. At the high level of location (7.6 m away from the fountain), the results were similar. When the fountain was OFF, the DO was 4.75 mg/ L and when the fountain was ON, the DO reached 6.75 mg/L. Note that there was a difference for the center point between fountain OFF and ON. The DO changed from 4.75 to 6.2 mg /L respectively.
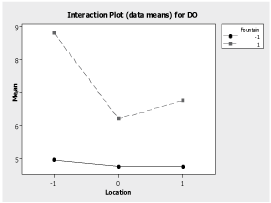
Figure 7 shows the interaction between fountain and time of day. There was an increase in DO at different times of day whether the fountain was ON or OFF. However, the magnitude of the increase depended on the fountain status. When the fountain was ON, the DO value increased from 7.06 mg /L at 7:00 am to 8.49 mg / L at 1:00 pm. When the fountain was OFF, the DO value changed from 4.75 mg / L to 4.85 mg / L. As with the interaction between fountain status and location, the center point responded differently when the fountain was ON compared to OFF mode. The accepted minimum level of dissolved oxygen required for aquatic species is 6 mg/L ( Hondzo & Steinberger, 2002 ). Results indicated that the floating fountain achieved the minimum desired level of dissolved oxygen.
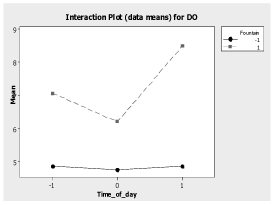
Cost Analysis of the Floating Fountain
Table 4 lists the materials, labor and maintenance cost for the floating water fountain system. All cost items have been rounded to the nearest $50. Small-scale solar PV installations require minimum yearly maintenance, if any. Thus, a lifetime maintenance cost of $1,000 is included.
Description | Cost ($) | Subtotal ($) |
---|---|---|
Materials | 5,000.00 | |
Modules | 3,000.00 | |
Pumps | 300.00 | |
Structure | 500.00 | |
Tank | 300.00 | |
Plumbing | 100.00 | |
Compressor | 200.00 | |
Batteries | 200.00 | |
Hoses and fittings | 150.00 | |
Linear current booster | 150.00 | |
Wiring | 100.00 | |
Labor | 600.00 | 600.00 |
Maintenance | 1,000.00 | 1,000.00 |
Total | 6,600.00 |
Total Cost = Materials Cost + Labor Cost + Maintenance Cost = $6,600.00 (4)
On the other hand, assuming that the fountain is powered entirely by utility grid electricity, the total estimated amount of Kw used during operation of the Floating Fountain is as follows:
Fountain:
(24 V x 8.4 Amp) x 24 hours = 4.8 kw-hr/day (5)
Aerator:
(12 V x 6.4 Amp) x 24 hours = 1.8 kw-hr/day (6)
The Total usage is 6.4 kw-hr/day.
Thus, the estimated annual output in kw-hr/year is equivalent to 2340 kw-hr/year
If the cost of electricity (utility) = $ 0.2175 / kw-hr (for Tallahassee, FL) = approximately $500 / year
(assuming no inflation and no increase in electricity tariffs)
The use of grid electricity will require all the materials listed in Table 4, with the exception of the PV modules and batteries. Since the cost of solar PV modules and batteries is $3,200, the above analysis yields a payback period of 3,200 / 500 = 6.4 years, which is economically profitable, considering that the useful life of photovoltaic modules is 20 — 30 years. There is also the added environmental benefit of using solar energy.
Conclusion
Solar-powered appliances present unique advantages over traditional devices. The Solar-Powered Water Fountain (SPOWF) system manufactured and tested in this work achieved its main function, i.e., aeration of a selected water body with the aid of the aerator and fountain. Consequently, the dissolved oxygen (DO) concentration was increased significantly from a low level of 4.5 mg/L to a high of 9.95 mg/L, an increase of about 120%. Statistical analysis of the data (DO measurements) was conducted using a Split Plot Design. This mathematical model described a linear relationship between the primary operating factors, location of the aerator and time of the day, and the output, DO.
Economic analysis conducted using a payback period approach, by comparing the solar generated power with the utility grid electricity, yielded a payback period of 6.4 years. Considering that photovoltaic systems have a useful life of between 20 to 30 years, this payback period is profitable both economically and environmentally, considering the enormous benefits of the aerator / fountain unit to aquatic life.
The SPOWF system has immense potential commercialization opportunities. Possible consumer markets include environmental, building and construction, parks and gardens, private homes, estate developers, aquatic, and energy conservation.
Acknowledgement
This research was made possible with funding support provided by the US Department of Education Title III Grant Programs for Minority Students. Special thanks go to Dr. Yaw A. Owusu, Director of the Research for Center for Cutting-Edge Technologies and Professor of Industrial and Manufacturing Engineering at the FAMU - FSU College of Engineering, Tallahassee, Florida, for his guidance during this work. Our gratitude also goes to Dr. Ibrahim Braimah, also of the Research Center for Cutting-Edge Technologies, for the initiative he took in the design and assembly of the solar-powered floating fountain.
Dr. Hans Chapman is an Assistant Professor at the Department of Applied Engineering and Technology, Morehead State University, Kentucky. He is a member of the Gamma Mu Chapter of Epsilon Pi Tau.
Mr. Eduardo Gomez obtained his MS in Industrial Engineering from Florida State University, Tallahassee, Florida.
Dr. Nilesh N. Joshi is an Assistant Professor at the Department of Applied Engineering and Technology, Morehead State University, Kentucky.
Dr. Sanjeev Adhikari is an Assistant Professor of Civil Engineering and Construction Management at the Department of Applied Engineering and Engineering Technology, Morehead State University, Kentucky.
References
Boyd, C. E. (1997). Advances in pond aeration technology and practices . INFOFISH Int., 2/97, pp. 24–28.
Braimah, I. (2004). Design and manufacture of affordable floating solar-powered water fountain (published master’s thesis). Department of Industrial and Manufacturing Engineering, Florida Agricultural and Mechanical University.
Butler, R. & Sinton, W. (2004). Solar-powered water pumping system in New York State . New York State Energy Research and Development Authority.
Dunlap, J. (2010). Water pumping applications . Photovoltaic Systems, American Technical Publishers, Orlando Park, IL, pp. 10-11.
Gomez, E. (2006). Performance and cost analysis of solar–powered water fountain (SPOWF) using a scaled model (published master’s thesis). Department of Industrial and Manufacturing Engineering, Florida Agricultural and Mechanical University.
Hondzo, M. & Steinberger, N. (2002). A semi-analytical model for dissolved oxygen mass transfer coefficient at the sediment–water interface . Hydrobiologia, 479 (1-3), pp. 63–68.
Lynch, J. & Commer, W. (1994). Understanding pond stratification . Ohio State University Extension Fact Sheet (OSUEFS), A-7-10-03. Retrieved from http://ohioline.osu.edu/a-fact/0007.html
Markvart, T. (1999). Solar electricity . New York: John Wiley & Sons.
Marshall, J. M. & Dimova-Malinovska, D. (2002). Photovoltaic materials–properties, technology and applications . NATO Science Series II: Mathematics, Physics and Chemistry, (80), pp.15-18.
Michaud, J. P. & Noel, S. (1991). A citizen’s guide to understanding and monitoring lakes and streams . Envirovision Environmental Consulting Service, Olympia, WA, 8-56.
Montgomery, D. C. (2005). Design and analysis of experiments . New York: John Wiley & Sons.
Myers, R. & Montgomery, D. C. (2002). Response surface methodology: process and product optimization using designed experiments (2nd ed.). New York: John Wiley & Sons.
Simpson, J. R., Kowalsky, S. M. & Landman, D. (2004). Experimentation with randomization restrictions: targeting practical implementation . Quality and Reliability Engineering International, 20 (5), pp. 481-495.
Wieland, A., & Kühl, M. (2006). Regulation of photosynthesis and oxygen consumption in a hypersaline cyanobacterial mat (Camargue, France) by irradiance, temperature and salinity . Journal of Microbiology and Ecology, 55 (2), pp. 195–210.
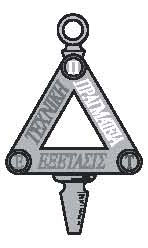